Introduction
The Eastern eel (Anguillia japonica) is one of 19 species within the freshwater eel family Anguillidae. They are common across the world, especially in East and South East Asian countries, predominantly in South Korea, China, Japan, Thailand, and Philippines (Seo et al., 2013). They are used in many different ways including consuming as food and using in traditional Chinese medicine to cure skin diseases (e.g., atrophoderma) and hemorrhoids (Ekanayake et al., 2005). It is well known that fish and fishery products represent a valuable source of nutrients of fundamental importance for diverse and healthy diets. Fish provides high-value protein, polyunsaturated omega-3 fatty acids (docosahexaenoic acid and eicosapentaenoic acid), and a wide range of essential micronutrients, including various vitamins (D, A, and B), minerals (e.g., calcium, iodine, zinc, iron, and selenium) (FAO, 2012). In addition to their high nutritious value, studies have been identified fish meat as a rich source of bioactive peptides with potentially valuable nutraceutical and pharmaceutical properties (Elavarasan et al., 2014; Halim et al., 2016). Bioactive peptides could be released from inactive original protein by enzymatic hydrolysis. These fish protein-derived bioactive peptides exhibit various biological activities such as antioxidative, anti-diabetic activities, anti-hypertensive, anti-coagulant, anti-proliferative, anti-obesity, and calcium-binding (Ngo et al., 2012). Moreover, it has been demonstrated that protein hydrolysates exhibiting antioxidative potential may contain peptides with other biological activities such as angiotensin I-converting enzyme (ACE) inhibitory activity (Samaranayaka et al., 2010).
Antioxidants are very important to human health. They can inhibit the oxidation reaction that cause cell damage or death in human body by removing free radical intermediates. Therefore, it is believed that antioxidants can decrease the risk of developing more than 100 diseases, including diabetes, heart disease, cancer, HIV (AIDS), stroke, and atherosclerosis, and then can slow the aging process (Ekanayake et al., 2005). Many natural antioxidants originating from plant and fish sources have been identified as free radical active scavengers (Clarke et al., 2013; Elavarasan et al., 2014; Mandal et al., 2009; Zhou et al., 2012). Hypertension, a long-term medical condition in which the blood pressure in the arteries is persistently elevated, is a major risk factor for cardiovascular disease. ACE plays a vital physiological role in the regulation of blood pressure. ACE cleaves angiotensin I to the potent vasoconstrictor angiotensin II and inactivates the vasodilator bradykinin (Ghanbari el al., 2015). These functions of ACE increase blood pressure and finally lead to hypertension. In addition, angiotensin II produced by ACE increases oxidative stress, inducing the production of reactive oxygen species (ROS) under high blood pressure conditions (Schiffrin and Touyz, 2004). Therefore, the inhibition of ACE activity or the blockade of angiotensin II formation may enhance the antioxidative system.
A number of studies have reported the presence of bioactivities in enzymatically hydrolyzed fish proteins from salmon (Salmo salar) (Darewicz et al., 2014), rainbow trout (Oncorhynchus mykiss) (Kim and Byun, 2012), bigeye tuna (Thunnus obesus), dark muscle (Qian et al., 2007), and chum salmon (Oncorhynchus keta) (Ono et al., 2003). Recently, there are several published studies reporting the presence of bioactivities in enzymatically hydrolyzed Asian swamp eel (Monopterus sp.) proteins (Azemi et al., 2017; Baharuddin et al., 2016; Halim et al., 2017; Halim et al., 2018; Jamil et al. 2016). They report that Asian swamp eel protein hydrolysate using alcalase have the antioxidant activity (Azemi et al., 2017), ACE inhibitory activity (Azemi et al., 2017; Baharuddin et al., 2016) and anticancer activity (Halim et al., 2018). However, there was little known about the potential for multiple bioactivities of protein derived from Eastern eel (A. japonica). The main objective of the present study was to evaluate and compare the antioxidative potential and ACE inhibitory activity of enzymatic hydrolysates and hot water extracts of Eastern eel (A. japonica).
Materials and methods
Hydrogen peroxide (H2O2), 1,1’-diphenyl-2-picrylhydrayl (DPPH), thiobarbaturic acid (TBA) and angiotensin I-converting enzyme (ACE) were purchased from Sigma-Aldrich (St. Louis, MO, USA). Ethylene diamine tetra-acetic acid (EDTA), 2,2’-azino-bis (3-ethylbenzthiazolin)-6-sulfonic acid (ABTS), iron sulphate (FeSO4·7H2O), peroxidase, 2-deoxyribose, monopotassium phosphate (H2HPO4), potassium diphosphate (KH2PO4), hydrochloric acid (HCl), vitamin C and pyrogallol were purchased from Fluka Co. (St. Louis, MO, USA). All the other reagents used were either extra pure or analytical grade. Alcalase® (Alcalase 2.4L) and Promatex® were obtained from Novozymes (Copenhagen, Denmark).
Eastern eel (Anguilla japonica) were collected from local reservoirs (Chilbo-susan, Jeongeup, Korea), frozen immediately with the help of fishermen, and thawed rapidly below 35°C for analysis. The fish meat and the fish whole body of eel including the frame, dark muscle, cut offs, viscera, skin, scales, bones and fins, were collected for the hot water extraction of eel. These were minced and mixed with water in the ratio of 1:10 (fish mince: water). The extraction was done on 100°C with reflux, where samples were collected on different hours (0, 5, 10, 15, 20 and 25 h). For the preparation of enzymatic hydrolysates, the fish meat was minced, mixed with the pre-warmed 0.1 M sodium phosphate buffer (pH 7) in the ratio of 1:10 (fish mince: sodium phosphate buffer) and each protease was added as the final conc. of 1.15%. The hydrolysis reaction was performed at 50°C for 10 h. The hydrolysates were collected at 2 hours’ interval (0, 2, 4, 6, 8 and 10 h). The collected hydrolysates were inactivated in boiling water for 10 min. After cooling at room temperature, each sample was clarified by centrifugation at 3,000 rpm for 20 min to remove any residue and stored at −20°C. Hydrolysates were designated as ME-ALP (Alcalase®), ME- PRH (Promatex®) and ME-APH (Alcalase® + Promatex®) based on the enzyme used for hydrolysis. Hot water extracts based on the source for extraction, were expressed as ME (meat extract) and WBE (whole body extract).
Moisture and ash contents of Eastern eel (Anguilla japonica) were determined according to the AOAC (2005), standard methods 930.15 and 942.05, respectively. Total nitrogen content was determined by using the Kjeldahl method according to AOAC (2005) method number 984.13. Crude protein was estimated by multiplying total nitrogen content by the factor 6.25. Crude fat was determined gravimetrically after Soxhlet extraction of samples with hexane. All measurements were performed in triplicate.
The protein contents of the hot water extracts and hydrolysates were estimated by the Lowry assay (Lowry et al., 1951). According to this procedure, the samples were first pretreated with copper ions in an alkali solution. The aromatic amino acids in the treated samples reduced the phosphomolybdic-phosphotungstic acid present in the Folin reagent. Since the endpoint of the reaction has a blue color, the amount of protein in the sample were estimated by reading the absorbance using a spectrophotometer (Optizen 3220 UV-VIS spectrophometer, Daejeon, Korea) at 750 nm. BSA was used as standard.
Determination of DPPH radicals scavenging activity was estimated with the modification of the methods used by Khan et al. (2012). A 100 μL of 0.4 mM DPPH solution and 100 μL of the sample were added, mixed well in 96 microwell plates for 10-20 seconds and incubated for 30 min at room temperature in the dark. After incubation, the absorbance was measured at 540 nm against the corresponding blank solution (ethanol without the sample). The control was prepared by taking 100 μL DPPH and 100 μL of distilled water. Percentage inhibition of free radical DPPH was calculated based on following equation.
Where Ac is the absorbance of control; As is the absorbance of mixture containing sample; Ab is the absorbance of blank.
ABTS radical-scavenging activity was determined by ABTS assay as described by Binsan et al. (2008). The working solution was prepared by mixing the two stock solutions (7 mM ABTS solution and 2.45 mM potassium persulfate) in equal quantities and allowing them to react for 12-16 h at room temperature in the dark. ABTS solution was then diluted by mixing distilled water to obtain an absorbance of 0.7-0.8 at 734 nm using a spectrophotometer (Optizen 3220 UV-VIS Spectrophotometer, Gyeonggi-do, Korea). 180 μL ABTS solution and 20 μL of the sample were mixed, left at room temperature for 2 min in the dark and read at 734 nm. ABTS radical-scavenging activity was calculated by the following equation.
Where Ac is the absorbance of control (ABTS and distilled water); As is the absorbance of mixture containing sample; Ab is the absorbance of blank (distilled water).
Ability of the samples to inhibit the autoxidation of pyrogallol was done by modifying Marklund and Marklund (1974). Briefly, 0.3 mL of the sample and 2.65 mL of 50 mM phosphate buffer (pH 7.4 at 37°C) containing 1 mM Na2EDTA were mixed in a cuvette. Freshly prepared 50 μL of 60 mM progallol in 1 mM HCl was added and the inhibition of pyrogallol autoxidation was measured at 325 nm using spectrophotometer. Absorbance of sample was recorded at 0 min and after 5 min and the increment of absorbance was calculated by the difference.
Where ΔAs is the absorbance increment of the mixture containing the sample and ΔAc is absorbance increment of the mixture without the sample.
The •OH scavenging activity was determined by modified methods of Chung et al. (1997). A 100 μL sample and 500 μL of 0.1 M phosphate buffer (pH 7.4) were mixed with 100 μL of 10 mM FeSO4, 100 μL of 10 mM EDTA and 100 μL of 10 mM 2-deoxyribose in order to make a total volume of 900 μL, then 100 μL of 10 mM of H2O2 were added, and incubated at 37°C for 4 h. After incubation, 0.5 mL of 2.8% TCA and 0.5 mL of 0.1% TBA dissolved in water were added to the reaction mixture and kept in a boiling water bath for 10 min. The absorbance was measured at 532 nm.
Where Ac is the absorbance of mixture without sample; As is the absorbance of mixture containing sample.
The H2O2 scavenging activity was determined according to the method of Muller (1985). The 20 μL of sample was dissolved in 100 μL of 0.1 M phosphate-buffered saline (pH 5.0), mixed with 20 μL of 10 mM H2O2, and incubated at 37°C for 5 min. Then, 30 μL of 1.25 mM ABTS and 30 μL of peroxidase (1 unit/mL) were added to the mixture and re-incubated at 37°C for 10 min. The incubation of ABTS with peroxidase resulted in the production of the radical ABTS+, which is blue green and absorbance of each sample was measured at 405 nm.
ACE inhibitory activity was measured according to the method described by Cushman and Cheung (1971) with some modification. This method was based on liberation of hippuric acid (HA) from hippuryl-l-histidyl-l-leucine (Hip-His-Leu, HHL) catalyzed by the ACE. The sample (25 μL) was pre-incubated with 50 μL substrate solution (8.3 mM HHL in 50 mM sodium borate buffer containing 0.5 M NaCl at pH 8.3) at 37°C for 10 min. The reaction started by adding 50 μL of pre-heated ACE (8 mU) solution in sodium borate and the mixture was incubated for 30 min at 37°C. The reaction was stopped by addition of 125 μL 1 N HCl. The formed HA was extracted with 750 μL ethyl acetate and centrifuged (3,000 rpm, 15 min). The organic phase (500 μL) was evaporated at 50°C for 4 h. The dried residue was dissolved in 750 μL of pre-heated water (60°C), vortexed for 5 min and the absorbance was read at 228 nm in a spectrophotometer (Optizen 3220 UV-VIS spectrophotometer, Korea). The extent of ACE inhibition was calculated using the following formula:
Where Ac is the absorbance of control (HHL and ACE), As is mixture containing sample, and Ab is blank (distilled water). The antihypertensive agent, captopril was used as a standard.
The sample preparation was carried out by hydrolysis with 6 M HCl at 110°C for 24 h and derivated by using phenyl isothiocyanate prior to HPLC analysis. The total amino acids compositions were analyzed by an automated amino acid analyzer (L-8900; Hitachi High-Technologies Corp., Tokyo, Japan).
All tests were conducted in triplicates. All results were expressed as mean±SD. The data was statistically analyzed by one-way ANOVA followed by Duncan’s multiple range tests using SPSS package program (Version 26, IBM SPSS statistics, Armonk, NY, USA). p-value less than 0.05 were considered statistically significant.
Results
According to the chemical composition results, Eastern eel contains 61.8% moisture, 14.8% protein, 20.7% fat, 1.5% ash, and 1.2% carbohydrate. In particular, the fat and protein contents are very high in Eastern eel. Excluding the moisture content, the ratios of fat and protein were 54.2% and 38.7% on a dry basis.
To monitor the degree of protein extraction, the protein concentrations of ME and WBE were determined at intervals of 5 h over an extraction time of 25 h (Fig. 1A). The initial concentrations in the ME and WBE were 0.364 and 0.116 mg/ml, respectively. The final protein concentrations in the ME and WBE were 5.08 and 6.87 mg/mL, respectively, corresponding to 14.1- and 59.1-fold increases from the initial protein concentration, indicating that the protein yield of the WBE was higher than that of the ME. The protein concentration curves of the hydrolysates tended to have an initial rapid phase during the first 2 h followed by a phase of slow increase after the core proteins had been hydrolyzed (Fig. 1B). The initial average protein concentration of eel meat was 0.147 mg/mL. The final protein concentrations in the ME-ALP, ME-PRH, and ME-APH were 10.6, 11.22, and 12.1 mg/mL, respectively, corresponding to 71.9-, 76.3-, and 82.2-fold increases from the initial protein concentration for ME-ALP, ME-PRH, and ME-APH, respectively. All hydrolysates showed higher protein yields than the eel extracts. At the same concentration, the ME-APH showed greater proteolytic activity.
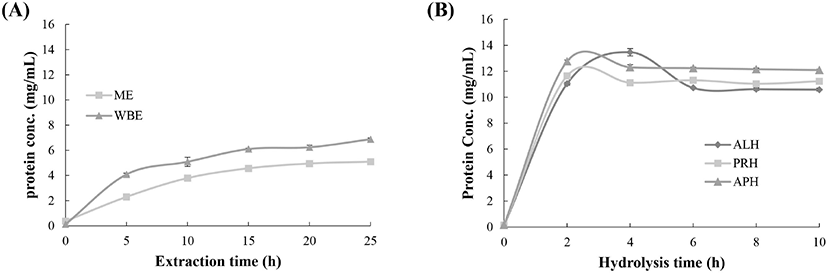
While the ME and WBE at 25 h did not show DPPH radical scavenging activity, the ME-APH was found to have greater scavenging ability (56.6%) followed by the ME-ALP (47.0%) and ME-PRH (30.1%) at 10 h (Fig. 2A; p<0.05). The changes in DPPH radical scavenging activity were monitored during the hydrolysis process, as shown in Fig. 2B. The highest level of activity in the ME-ALP was 57.0% at 2 h; that in the ME-APH and ME-PRH was 59.9% and 48.1% after 8 h of hydrolysis, respectively.
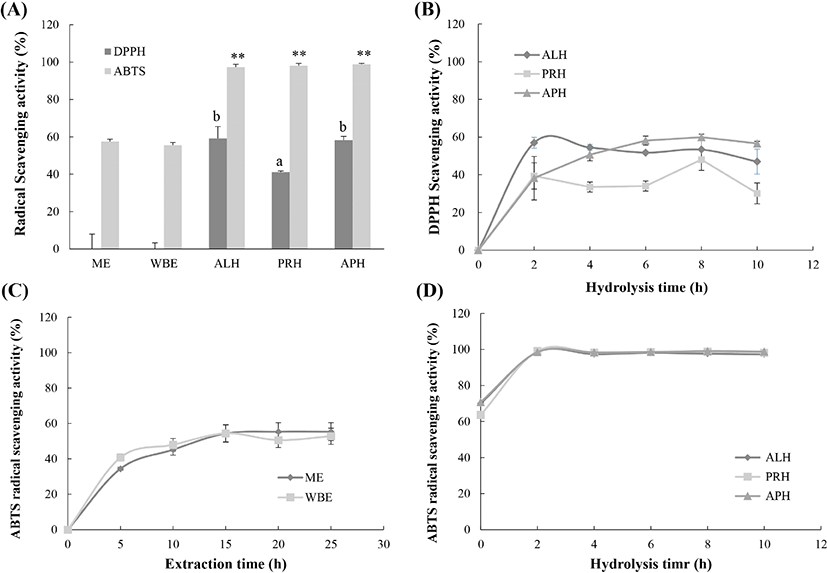
As shown in Fig. 2A, the ABTS radical scavenging activities of the ME and WBE were 55.5% and 57.5% at 25 h, but they were not significantly different (p=0.169). All hydrolysates showed an ABTS radical scavenging power of > 98%. ABTS radical scavenging activities higher than 50% were recorded after 15 h of extraction (54.5% at 15 h for the ME and 55.4% at 20 h for the WBE; Fig. 2C), while all hydrolysates reached > 98% ABTS radical scavenging power at 2 h. The ME-PRH (99.1%) at 2 h had the highest level of activity, followed by the ME-APH (99.03%) at 8 h and ME-ALP (98.50%) at 2 h (Fig. 2D).
The highest O2− scavenging activities of the eel hot water extracts were recorded as 41.0% at 5 h for the ME and 28.7% at 20 h for the WBE (Fig. 3A), respectively. The O2− scavenging activity of the eel hydrolysates increased rapidly to 40% within 2 h of hydrolysis and slowly settled to approximately 65% at 10 h. The O2− scavenging activities of the hydrolysates at the end of hydrolysis (10 h) were recorded as 59.9% for the ME-ALP, 67.0% for the ME-PRH, and 64.5% for the ME-APH. The highest level of O2− scavenging activity was recorded at 10 h for the ME-PRH (67.00%) (Fig. 3B).
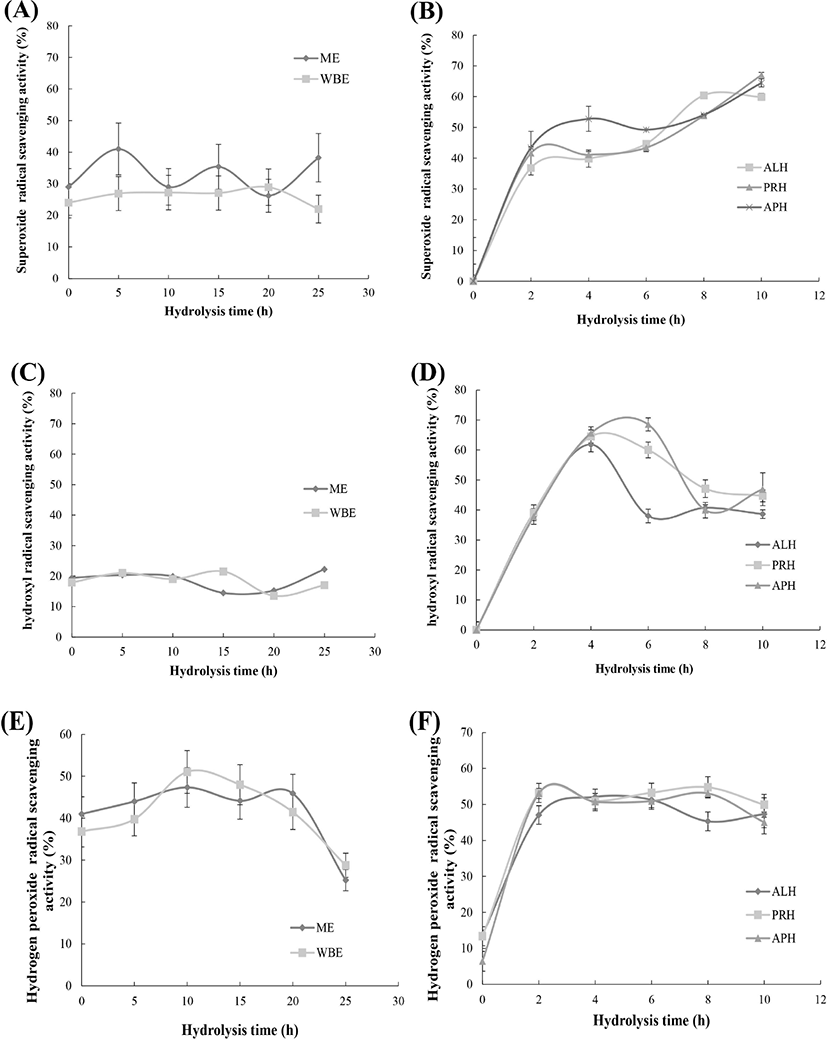
The greatest •OH scavenging activities observed in the hot water extracts were 22.4% at 25 h and 21.5% at 15 h for the ME and WBE (Fig. 3C), respectively; those in the hydrolysates were 61.8% and 63.9% at 4 h for the ME-ALP and ME-PRH, and 68.0% at 6 h for the ME-APH (Fig. 3D).
The highest H2O2 scavenging activities were 47.4 % at 10 h for the ME and 51.1% at 10 h for the WBE (Fig. 3E). The highest H2O2 scavenging activities in the hydrolysates were 52.6% after 4 h of hydrolysis for the ME-ALP, and 55.4% and 53.8% at 8 h for the ME-PRH and ME-APH (Fig. 3F).
The hydrolysates showed similar trends in the DPPH radical, ABTS radical, O2−, •OH, and H2O2 scavenging assays in terms of their biological activities. Thus, the IC50 values (concentration required to scavenge 50% of radical, anion, or H2O2) of the hydrolysates (10 h) were compared in each assay. Ascorbic acid and α-tocopherol were used as positive controls. As shown in Table 1, the lowest IC50 value of DPPH was 2.73 mg/mL in the ME-ALP; that of α-tocopherol was 0.36 mg/mL. However, the lowest IC50 values from the ABTS scavenging activity assay was found in the ME-PRH with 1.13 mg/mL. The lowest IC50 values from the •OH and H2O2 scavenging activity assays were also found in the ME-PRH, at 1.11, and 1.3 mg/mL, respectively. The IC50 values of O2− scavenging activity were 3.01, 3.07, and 3.14 mg for the ME-ALP, ME-PRH, and ME-APH, respectively, which are not significantly different. The IC50 value of ascorbic acid was 0.22 mg/mL for ABTS radical scavenging activity, 0.31 mg/mL for H2O2 radical scavenging activity, and 0.42 mg/mL for •OH scavenging activity. The ME-PRH exhibited the highest ABTS, •OH, and H2O2 scavenging activities, while the ME-ALP exhibited the highest DPPH scavenging activity.
The ACE inhibitory activities of the hot water extracts at 25 h were 8.6% for the ME and 0% for the WBE, while those of the hydrolysates at 10 h were approximately 75% for all hydrolysates (72.6% for the ME-ALP, 75.7% for the ME-PRH, and 75% for the ME-APH; Fig. 4A). The ACE inhibitory activities of the hydrolysates at 10 h of hydrolysis were not significantly different (p=0.108). The ACE inhibitory activities of the hydrolysates showed an initial rapid increase during the first 2 h of hydrolysis and then maintained a similar activity for all hydrolysates. The highest activity levels were 74.79% (8 h) for the ME-ALP, 78.30% (8 h) for the ME-PRH, and 80.42% (2 h) for the ME-APH (Fig. 4B). To characterize the ACE inhibitory activity of the hydrolysates further, IC50 values for ACE inhibition of the hydrolysates were determined as shown in Table 1. The IC50 values for the ME-ALP, ME-PRH, and ME-APH were 132.91, 110.37, and 115.17 μg/mL, respectively (p=0.164). The IC50 value for captopril, a synthetic ACE inhibitor (positive control), was 0.0567 μg/mL. Similar to the results for antioxidative activity, the greatest ACE inhibitory activity was found in the ME-PRH, although the values were not significantly different among the hydrolysates.
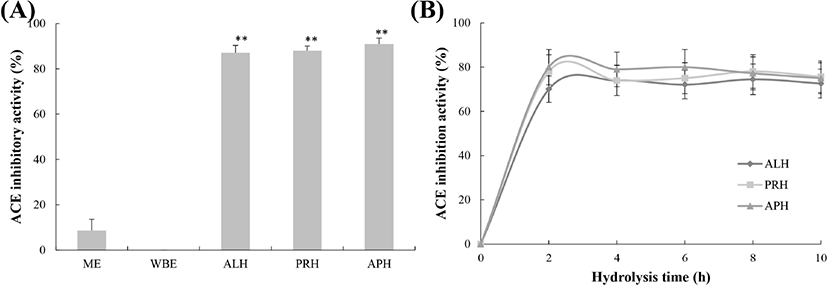
The amino acid composition of eel meat extract and hydrolysates were compared in Table 2. The contents of glycine and alanine were to approximately 37% and the ratio of hydrophobic amino acids to total amino acids was 0.2081 on ME. On three hydrolysates of Eastern eel, the contents of leucine, valine and tyrosine, which were hydrophobic amino acids, were increased more than 2 times and glycine and alanine were decreased to 8.46 and 8.56%, respectively. The ratios of hydrophobic amino acids to total amino acids for the ME-ALP, ME-PRH, and ME-APH were 0.404, 0.3992, and 0.4004, respectively, indicating the hydrophobic amino acids on three hydrolysates of Eastern eel were released via enzymatic hydrolysis.
Discussion
The eels were considered as a nutritious and tasty species, and as an oriental medicine (Khanh and Ngan, 2010). Especially in Korea and Japan, eels were thought to boost stamina in hot weather, and therefore they have been consumed as the various forms including hot water extracts. Recently, fish protein hydrolysates are widely focused as the main source of bioactive peptides for various health benefits. In this study, to evaluate the health benefits of Eastern eel extracts and hydrolysate, the antioxidative and ACE inhibiting effects of them were compared.
An efficient way of assessing antioxidative activity of eel extracts and hydrolysates is to examine the ability to trap the free radicals produced in various multiple reactions. The radical system used for evaluation of antioxidative activity may influence the experimental result, because of differences in the mechanism of antioxidative action being measured or the reaction conditions used. Therefore, two or more radical systems are required to investigate radical scavenging capacities (Yu et al., 2002). A range of assays, including DPPH, ABTS, O2−, •OH, and H2O2 scavenging activities, were performed to assess the antioxidative activities of the eel extracts and hydrolysates. These trapping techniques measure the radical scavenging activities of samples against free radicals such as DPPH, ABTS, O2−, •OH, and H2O2.
DPPH is a stable free radical molecule that changes color from deep violet to colorless or pale yellow on reduction by either hydrogen or electron donation. Substances that can donate hydrogen or electrons are considered to have anti-oxidative activity. The blue/green ABTS•+ radical cation produced through the reaction between ABTS and potassium persulfate is converted to a colorless neutral form by a reaction with antioxidants (Carocho and Ferreira, 2013). O2− is normally formed during the first step of a cellular oxidation reaction, and its effect can be magnified as it produces other kinds of cell-damaging free radicals and oxidizing agents (Liu and Ng, 2000). •OH is the neutral form of the hydroxide ion (OH−) and the most ROS in biological systems. This radical can damage all types of macromolecules, including carbohydrates, nucleic acids, lipids, and amino acids, and it has the strongest cell-damaging action among free radicals (Chung et al., 1997; Liu and Ng, 2000). In addition, this radical combine with the nucleotides in DNA and cause strand breakage, leading to carcinogenesis, mutagenesis, and cytotoxicity (Khan et al., 2012). H2O2 is a non-reactive but unstable molecule. Sometimes it is converted to free OH− radicals, which react with the proteins, membrane lipids, and DNA in living cells and cause tissue damage and cell death (Khan et al., 2012).
In our present study, the antioxidative activities of the eel hydrolysates were higher than those of extracts in all types of antioxidative assay systems. These results are in line with Slizyte et al.’s report (2016), showing that the fish protein hydrolysates from defatted salmon backbones obtained with commercial enzymes increased the antioxidative DPPH radical scavenging ability by 10-25% compared to those without hydrolysis. Among eel hydrolysates, especially the eel hydrolysate treated with Protamex (ME-PRH) exhibited the highest ABTS, •OH, and H2O2 scavenging activities, indicating ME-PRH could be potential anti-oxidant against free radicals with the strong cell-damaging action.
ACE is a dipeptidyl carboxypeptidase (EC. 3.4.15.1) that was originally isolated from horse blood. It exists as a membrane-bound enzyme as well as a circulatory or globular enzyme (Ortiz-Salmeron et al., 1998). In the renin-angiotensin system, renin promotes the hydrolysis of angiotensin to the decapeptide angiotensin I and ACE hydrolyzes the dipeptide His-Leu at the C-terminus of angiotensin I to produce active angiotensin II, which has a vasoconstricting action. In addition, ACE hydrolyzes and inactivates the vasodilator bradykinin (Das and Soffer, 1975). Therefore, the inhibition of ACE will prevent the production of angiotensin II and inhibit the hydrolysis of bradykinin, which will be useful for controlling high blood pressure and hypertension. In fact, ACE inhibitors are used to treat myocardial infraction, hypertension, and other cardio-related diseases. However, most ACE inhibitors are synthetic, and these drugs have certain adverse side effects (Raghavan and Kristinsson, 2009). Therefore, a number of studies have been conducted to find ACE inhibitors from natural resources, including food protein hydrolysates; the most useful natural ACE inhibitors were found to be peptides from protein hydrolysates (Iwaniak et al., 2014). Sila et al. (2013) recorded 87% ACE inhibition activity for an Alcalase® hydrolysate from the muscle of Barbel (Barbuscallensis), and Athukorala and Jeon (2005) recorded 85% for Alcalase® and 74% for Promatex® hydrolysates of different algal species. In our results, the measurements were very high (> 70%). The IC50 values for the ME-ALP, ME-PRH, and ME-APH were 132.91, 110.37, and 115.17 μg/mL, respectively. In Coscueta et al. (2016)’s reports, the potential antihypertensive peptides from milk and soy derived protein hydrolysates showed the IC50 values of 33-930 μg/mL, corresponding to the range of eel hydrolysates. In addition, Karaki et al. (1990) reported the anti-hypertensive effect in hypertensive rats using a casein hydrolysate with IC50 value of 166 μg/mL. The standard ACE inhibition peptides, Val-Trp and Ala-Tyr, were reported to have the ACE inhibition activity with IC50 value of 200-900 μg/mL (Sato et al., 2002, Slizyte et al., 2016). Baharuddin et al. (2016) reported the ACE inhibitory activity of eel (Monopterus sp.) protein hydrolysate with IC50 value of 2.128 mg/mL at DH 36%. These results were rather lower than ACE inhibition activities of Eastern eel hydrolysates treated with Alcalase®, Protamex®, and their mixture in this study, confirming that enzymatic hydrolysates of Eastern eel (A. japonica) could be excellent natural source for ACE inhibitor candidates.
Generally, it has been demonstrated that the biological activities of proteins can be increased via hydrolysis with various enzymes (Chen et al., 1995). During hydrolysis, native proteins are cleaved by enzymes, and hidden hydrophobic sites inside the proteins are exposed. These exposed hydrophobic amino acid residues in the peptides obtained confer various biological activities, especially antioxidative and ACE inhibitory activities (Faithong et al., 2010). Additionally, the biological activities of protein hydrolysates depend on the protein substrate, the specificity of the enzyme, the conditions used during proteolysis, and the degree of hydrolysis (Sila et al., 2013). Elavarasan et al. (2014) reported that protein hydrolysates differ in their antioxidant properties based on the nature of the proteases used as the sequence of released peptides are different chiefly based on the type of enzyme used for cleavage. Protein hydrolysates produced by different enzymes have different-sized peptides and amino acid sequences, which can have significantly different biological activities (Centenaro et al., 2011). In this study, the observed antioxidative and ACE inhibitory activities may be related to the enzyme used and each hydrolysate’s composition. Alcalase® is a serine alkaline endoprotease with broad specificity; it hydrolyzes most peptide bonds, but preferentially those containing aromatic amino acid residues (Doucet et al., 2003). Promatex® is also an endopeptidase with broad specificity for hydrophobic amino acids (Ward, 1983). Therefore, each hydrolysate produced by theses enzymes may produce various hydrophobic peptides, which might stabilize radicals and inhibit ACE. Actually, Table 2 shows that hydrophobic amino acid contents on three hydrolysates of Eastern eel were higher than on meat extract, indicating the release of hydrophobic peptides via enzymatic hydrolysis. However, additional investigations are required to obtain more information about the amino acid sequences of the active peptides.
Collectively, the enzymatic hydrolysates of Eastern eel (A. japonica) by Alcalase® and Promatex® could be excellent natural source for antioxidant and ACE inhibitor candidates.