1. Introduction
Whipped cream is one of the dairy products commonly used in desserts, cakes, pastries, and ice creams. It has a fat content of approximately 30%-40% and is an oil-in-water (O/W) emulsion that produces foam through the whipping process (Li et al., 2020b; Sajedi et al., 2014). The properties and texture of whipped creams are influenced by several factors, including good whipping ability, overrun, stability, and firmness (Rezvani et al., 2020). The quality of whipped creams depends on the destabilization of fats for crystallization, as the foam structure of whipped cream is stabilized by partially coalesced fat globules (Wu et al., 2023; Yang et al., 2022). Partial coalescence only occurs when two or more fat globules collide to form crystalline links in a crystalline fat-containing O/W emulsion (Liu et al., 2021). Proteins and fat globules both contribute to the formation and stabilization of foam in whipped cream (Sajedi et al., 2014).
Recombinant plant-based creams have been widely used in the food industry, including dairy products, beverages, and confectionery, to address the issue of dairy products being unsuitable for some individuals, such as those with lactose intolerance and milk protein allergies (Fu et al., 2020). However, it is important to note that recombinant plant-based whipped creams are typically manufactured from hydrogenated vegetable fats, which contain trans fats and may increase the risk of cardiovascular disease, diabetes, and cancer (Cao et al., 2020; Islam et al., 2019). In comparison, cocoa butter and canola oil are natural fats obtained from cocoa seeds (Theobroma cacao) and canola from the Brassicaceae family.
Recently, there has been a growing interest in utilizing sustainable plant-based proteins as functional ingredients in foods due to consumer demand for “clean labels” (Moll et al., 2020; Zhu et al., 2018). This is in response to concerns about animal welfare and the increased greenhouse emissions associated with animal-based proteins (Kumar et al., 2022). Animal agriculture for animal-based protein is a leading cause of deforestation, freshwater utilization, eutrophication, and direct greenhouse gas emissions (Djekic et al., 2014; Theurl et al., 2020). Peas (Pisum sativum L.) are a good source of bioactive peptides due to their high nutritional value and lysine content. Peas are composed of 25% protein, 55%-68% starch, and 6.5% fiber (Burger and Zhang, 2019). Pea proteins can be classified into the following four groups: globulin, albumin, prolamin, and glutelin (Lu et al., 2020). At neutral pH, pea proteins are superior emulsifiers and foaming agents compared to soy proteins (Aluko et al., 2009). Therefore, they can effectively function as emulsifiers and foaming agents in plant-based whipped creams. The functional attributes of proteins, such as emulsification, foam formation, viscosity, and gelation, are closely related to their structural configurations (Di et al., 2022). Altering the structural framework of proteins can significantly enhance their functional efficacy (Sajedi et al., 2014). Therefore, to modify the structure of proteins, various methods including ultrasound, pH-shifting, alkali, and enzymes have been applied. Previous studies have shown that ultrasonic treatment significantly improves the solubility, water/oil holding capacity, foaming, and emulsifying capacity of pea protein (Zhao et al., 2022). Similarly, pH-shifting treatment at pH 11 has been found to significantly increase the solubility, emulsifying, and foaming properties of faba bean protein isolates (Alavi et al., 2021).
Ultrasound refers to pressure waves with frequencies above 20 kHz and is defined as sound waves with frequencies above the threshold of human hearing (>16 kHz) (Bariya et al., 2023; Muñoz et al., 2021). Ultrasonication of proteins can alter the physicochemical properties of various protein sources, including soy protein, pea protein, and egg white protein (OSullivan et al., 2016). Several recent studies have demonstrated that high-intensity ultrasound can improve the solubility, emulsification, foaming, and gelling properties of proteins (Zhu et al., 2018). Therefore, this study aims to investigate the quality characteristics of plant-based whipped creams based on ultrasonicated pea proteins and their potential to replace dairy whipped creams.
2. Materials and methods
Isolated pea protein (PPI) (Almi GmbH, Oftering, Austria), cacao butter (Europe Chocolate Company N.V., Westmalle, Belgium), and canola oil (Ottogi Co. Ltd., Anyang, Korea) were used as ingredients for the plant-based whipped cream. The PPI was contained of 80 g of protein, 3 g of carbohydrate, 8.3 g of fat and 2.2 g of salt per 100 g (Almi GmbH). The control used as a control was dairy whipping cream (Maeil Dairies Co. Ltd., Seoul, Korea). The dairy whipping cream was contained of 2 g of protein, 3.5 g of carbohydrate and 38 g of fat (Maeil Dairies Co. Ltd.).
The plant-based whipped cream is prepared using different types of proteins and fats from the control, as shown in Table 1. The samples using pea protein were named ultrasonication (UPP) and no ultrasonication (PP) depending on whether they were ultrasonicated or not.
Ingredients | Pea protein isolated (PPI) | Cacao butter | Canola oil | Water | Total |
---|---|---|---|---|---|
Weight (g) | 3 | 24 | 6 | 67 | 100 |
The protein solution was prepared by hydrating the PPI through magnetic stirring for 1 h at 20-30°C. After hydration, the UPP samples to be ultrasonicated were treated at an intensity of 360 W at a frequency of 24 kHz for 6 min using an ultrasonic machine (BKUP-900K, Bio Koncision Inc., Gwacheon, Korea) equipped with a 13 mm probe. The ultrasonic time used in this study was chosen based on the difference in protein properties, such as protein solubility, emulsifying activity index, and foam capacity, as well as whipped cream properties, including overrun and foam drainage. These results were obtained from our preliminary studies, as presented in Fig. S1, Fig. S2, and Fig. S3. Ultrasonication was performed with the probe submerged approximately 1.5 cm in the protein solution, and the samples were immersed in an ice water bath to prevent their temperature from rising during the treatment.
Cocoa butter and canola oil were dissolved in a water bath (JSIB-22T, JS Research Inc., Gongju, Korea) at 50°C for 30 min. The cream was prepared by mixing the hydrated protein solution with the dissolved oil phase using a hand mixer (HR2535/00, Philips, Amsterdam, Netherlands) at 14,900 rpm for 2 min The temperature was maintained at approximately 50°C. The cream was then aged for 24 h at 4°C.
Sample 100 g was refrigerated at 4°C for 24 h and then whipped using a digital hand mixer (LW-2003 A, Llantek Electrical Appliances Co. Ltd., Shenzhen, China) at stage 13, which provides 360 W.
The moisture content change was measured by weighing 3 g of the sample according to the normal pressure drying method, spreading it on an aluminum dish, and drying it in a 105°C dry oven (JSOF-100, JS Research Inc.) for 5 h, and subsequently cooling it in a desiccator for 30 min. Following measurement, the results were expressed as percentages (%) according to the following equation:
W0: the initial weight of the sample.
W1: the weight of the sample after drying.
The pH was measured using a pH meter (Orion 3 star, Thermo Electron Co., Waltham, MA, USA).
After whipping, whipped creams were stored in a petri dish (50×15 mm) at 4°C for 24 h before measuring the L* (lightness), a* (redness), and b* (yellowness) values of the surface using a colorimeter (CR-400, Konica Minolta Co., Osaka, Japan) calibrated with a standard white plate (L*=92.83, a* =−1.88, and b*=9.11). Each sample was measured five times, and the average value was shown.
The whipping time that produces the best results was determined by visually assessing the cream. The optimal whipping time is when the cream holds its shape and does not immediately flow around the wire (Zeng et al., 2021).
The method of Rezvani et al. (2020) was used to measure the overrun. To compare the masses for the same volume, the masses were compared by filling the same container. Excess cream was removed from the container using a metal scraper, and a constant volume of whipped cream was weighed. The overrun was calculated using the following equation:
Twenty grams of freshly whipped cream were placed in a funnel lined with microfiber paper (WM.1004110, Whatman Inc., Maidstone, UK) and placed in a thermostat (JSRH-150CP, JS Research Inc.) at 25°C for 4 h. The rate of foam drainage was calculated using the following equation (Rezvani et al., 2020):
Fat destabilization was measured using the turbidimetric method based on the method of Petrut et al. (2016). Two samples were used, one from unwhipped emulsion and one from whipped cream, diluted at a ratio of 1:300. The absorbance of each sample was measured at 540 nm using a spectrophotometer (Evolution 201, Thermo Fisher Scientific Inc., Madison, WI, USA). The amount of light absorbed depended on the size and number of fat globules dispersed in the diluent. The fat destabilization rate was calculated using the following equation:
Auw: the absorbance of the un whipped emulsion dilution.
Aw: the absorbance of the whipped cream dilution.
The optical microscope (Leica ICC50 HD, Leica Microsystems Ltd., Heerbrugg, Switzerland) was used to observe the bubble distribution and fat globule particle size in the whipped cream. The bubble distribution was observed at 40× and the fat globule particle size was observed at 400× and measured in 200 random samples using ImageJ (Version 1.8.0, U. S. National Institutes of Health, Bethesda, MD, USA).
Texture profile analysis was measured using a modified method of Gafour and Aly (2020). Whipped cream samples that were stored at 4°C for 4 h were placed in a 25 mL beaker and subjected to a two-bite compression test using a texture profile analyzer (Compac-II, Sun Scientific Co., Tokyo, Japan) equipped with a 20 mm diameter cylinder probe. The measurement conditions were as follows: table speed, 60 mm/min; load cell, 2 Kgf; distance, 3 mm; and clearance, 10 mm. The hardness, cohesiveness, springiness and adhesiveness of whipped cream were obtained.
The apparent viscosity of the whipped cream was measured at 4°C using a rheology rheometer (HR 10, TA Instruments, New Castle, DE, USA) based on the method of Yang et al. (2022). The samples’ apparent viscosity was measured at 1,000 μm intervals using a 20 mm plate at a constant temperature of 4°C and a shear rate of 0.1-100 s−1. Prior to measurement, all samples were equilibrated for 180 s, and 10 points per decade were measured per decade.
The viscoelastic properties of the whipped cream, including storage modulus (G′), loss modulus (G″), and tan δ, were measured using a rheology rheometer (HR 10, TA Instruments) based on the method of Yang et al. (2022). The measurements were taken at a constant temperature of 4°C and a frequency range of 0.1-100 rad/s (strain rate, 0.1%). Prior to measurement, all samples were equilibrated for 180 s, and 10 points per decade were measured per decade.
A sensory evaluation was conducted with 20 panelists, trained based on the method of Rezvani et al. (2020). Panelists assessed attributes such as appearance, color, flavor, firmness, and overall acceptability for each sample using a 7-point scale. Subjective indicators (1: very poor to 7: very good) were used for appearance, color, flavor, and overall acceptability, while objective indicators (1: very weak to 7: very strong) were used for firmness. The sensory evaluation was conducted safely and with final exemption from the Kyungpook National University Institutional Review Board (Approval number: KNU-2023-0485).
3. Results and discussion
The moisture contents, pH, and color values of the whipped creams are shown in Table 2.
The fat content of CON was 38%, while the plant-based pea protein whipped cream had a fat content of 30%, resulting in an approximate 8% difference in moisture content. No significant difference in the moisture content of the plant-based samples was observed since the same ingredient ratios were used.
No significant differences in pH levels were observed for any of the three samples, despite the CON and plant-based whipped creams having different ingredients. The plant-based samples did not differ, confirming that ultrasonication did not affect the pH level.
Depending on the proteins in the whipped cream, it is possible to change the colorimetry by modifying the size and surface properties of the particles, affecting light scattering, along with parameters that affect the partial coalescence and overrun of fat globules (Milovanovic et al., 2021). L* decreased in the order of CON, UPP, and PP. The volume of UPP increased due to its higher overrun compared to PP, resulting in an increase in L* (Balthazar et al., 2017). The order of decrease for a* was PP, CON, and UPP, which is consistent with the fat destabilization trend shown in Fig. 1. This is because fats, which are yellow in whipped creams, bind to proteins and become slightly green in color, thereby reducing their value (Rezvani et al., 2020). The increase in b* of whipped cream was in the order of CON, PP, and UPP. A significant difference was observed between dairy and plant-based pea protein whipped creams due to the effect of fat. The b* values were affected by carotenoids in milk fat for dairy whipped cream and cocoa butter for plant-based whipped creams (Samakradhamrongthai et al., 2021). Additionally, Table 3 shows that UPP is significantly more yellow than PP because UPP has smaller fat globules than PP (Stinco et al., 2012).
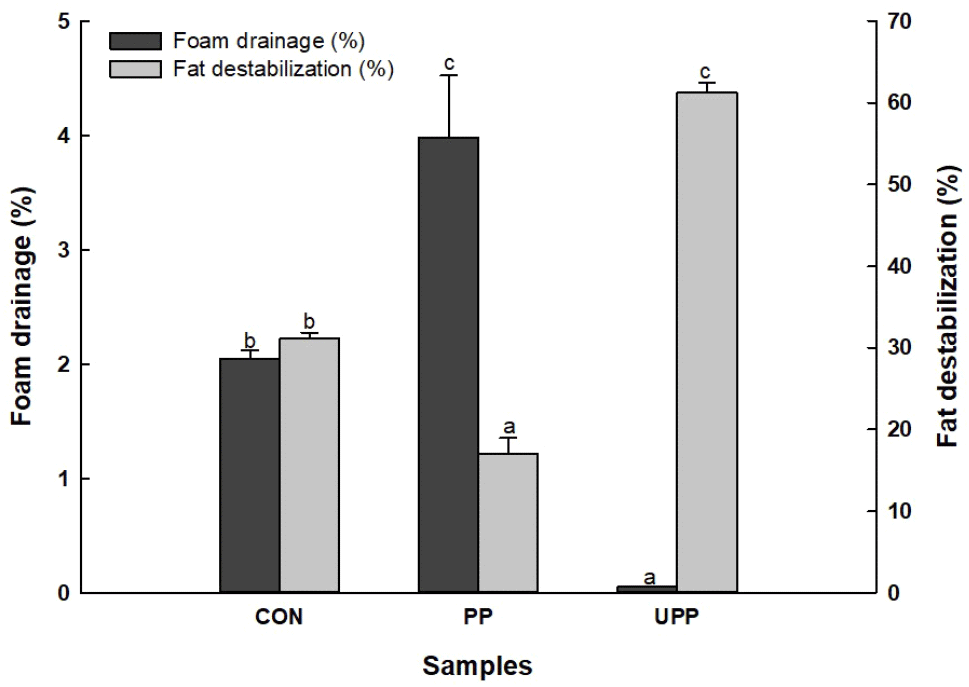
The whipping time and overrun of the whipped creams are shown in Table 3.
Whipping time is determined by the extent of partial coalescence of fat globules in whipping cream (Dhungana et al., 2020). Excessive whipping can cause the foam to collapse and form butter granules (Li et al., 2020a). The whipping time of UPP is significantly shorter than that of PP. Li et al. (2020b) reported that ultrasonication resulted in smaller protein particle sizes, leading to a thinner interfacial layer of proteins covering the surface of the fat globules, which facilitated faster partial coalescence and reduced whipping time. This confirmed that ultrasonication had a positive effect on the plant-based pea protein whipped cream.
Overrun is the percentage increase in the volume of whipped cream due to air entrapment during the whipping process and is the ability of the emulsion to trap air bubbles during whipping (Fu et al., 2020; Yang et al., 2022). Nguyen et al. (2022) reported that the overrun depends on the following two factors: surface-mediated partial adhesion, which refers to the connection between fat globules on the surface of the foam, and foam adsorption on the surface of proteins, which reflects the foam-holding capacity of the protein portion in the whipped cream. The overruns shown in Table 3 are significantly lower for UPP, CON, and PP, respectively. The results are consistent with previous studies where ultrasonication increased the overrun due to the improvement of protein foaming properties (Yang et al., 2022).
The foam drainage of the whipped cream is shown in Fig. 1. The foam drainage rate was used as an indicator to evaluate whipped cream stability (Dhungana et al., 2020). One of the main challenges in using whipped cream is the partial loss of cream serum during storage (Athari et al., 2021). Therefore, the cream with less drainage is more stable (Fu et al., 2020). According to Peng et al. (2018), the factors that affect foam stability are fat globules and protein. The results showed that PP had the highest drainage rate, which resulted in the worst foam stability. Conversely, UPP had a lower drainage rate than CON, confirming the superior foam stability of the ultrasonicated pea protein whipped cream. The ultrasonication process allowed pea proteins to be rapidly adsorbed at the air–water and fat–water interfaces, which reduced fat globule particle size and improved foam stability (Liu et al., 2023).
The fat destabilization of the whipped creams is shown in Fig. 1. Whipped creams require the partial coalescence of fat crystal globules to enable the destabilization process necessary for bubble emulsions to form bubble trapped crystal structures (Petrut et al., 2016; Yang et al., 2022). The partially coalesced fat globules form a network to support the bubbles created during the whipping process, which constitutes the desired texture and stability of the final product (Wang et al., 2022). During the whipping process, fat globules partially destabilize, and high fat coalescence can occur, resulting in a higher overrun of whipped creams (Fu et al., 2020). The degree of fat destabilization decreases in the order of UPP, CON, and PP. This trend is consistent with a previous study (Fu et al., 2020), as shown in Table 2. According to Liu et al. (2021), small fat crystal aggregates have a positive effect on fat globule coalescence. Table 3 shows that the fat destabilization degree of UPP is affected by the small fat globule particle size of UPP. Ultrasonication effectively dissociated protein subunits, which increased the protein adsorption content at the fat interface and promoted partial adhesion (Yang et al., 2022).
Fig. 2(A) shows the distribution of bubbles in the whipped cream at 40× magnification, and Fig. 2(B) shows the distribution of fat globules inside the whipped cream after whipping at 400×. The results indicate that CON and UPP have a high distribution of small bubbles, whereas PP retains larger bubbles compared with the other samples. This effect on bubble size is correlated with the fat destabilization and fat globule size (Liu et al., 2021). As shown in Fig. 2(B) and Table 3, fat globules are significantly increased in CON, UPP, and PP. It is noticeable that the fat globule particle size decreased more in UPP than that in PP after ultrasonication. The reduction in fat globules is likely due to the role of ultrasound-modified proteins, as the fat was not directly ultrasonicated in this study. Ultrasound can dissociate proteins into smaller units, which loosens their structure and enhances their interfacial adsorption activity at the fat-water interface, which can increase fat adhesion and affect fat particles (Silva et al., 2020; Yang et al., 2019; Zhong and Xiong, 2020). This study confirms that ultrasonication effectively reduces fat globule particle size, and ultimately, the smaller particle size positively affects foam drainage, making UPP the most stable whipped cream.
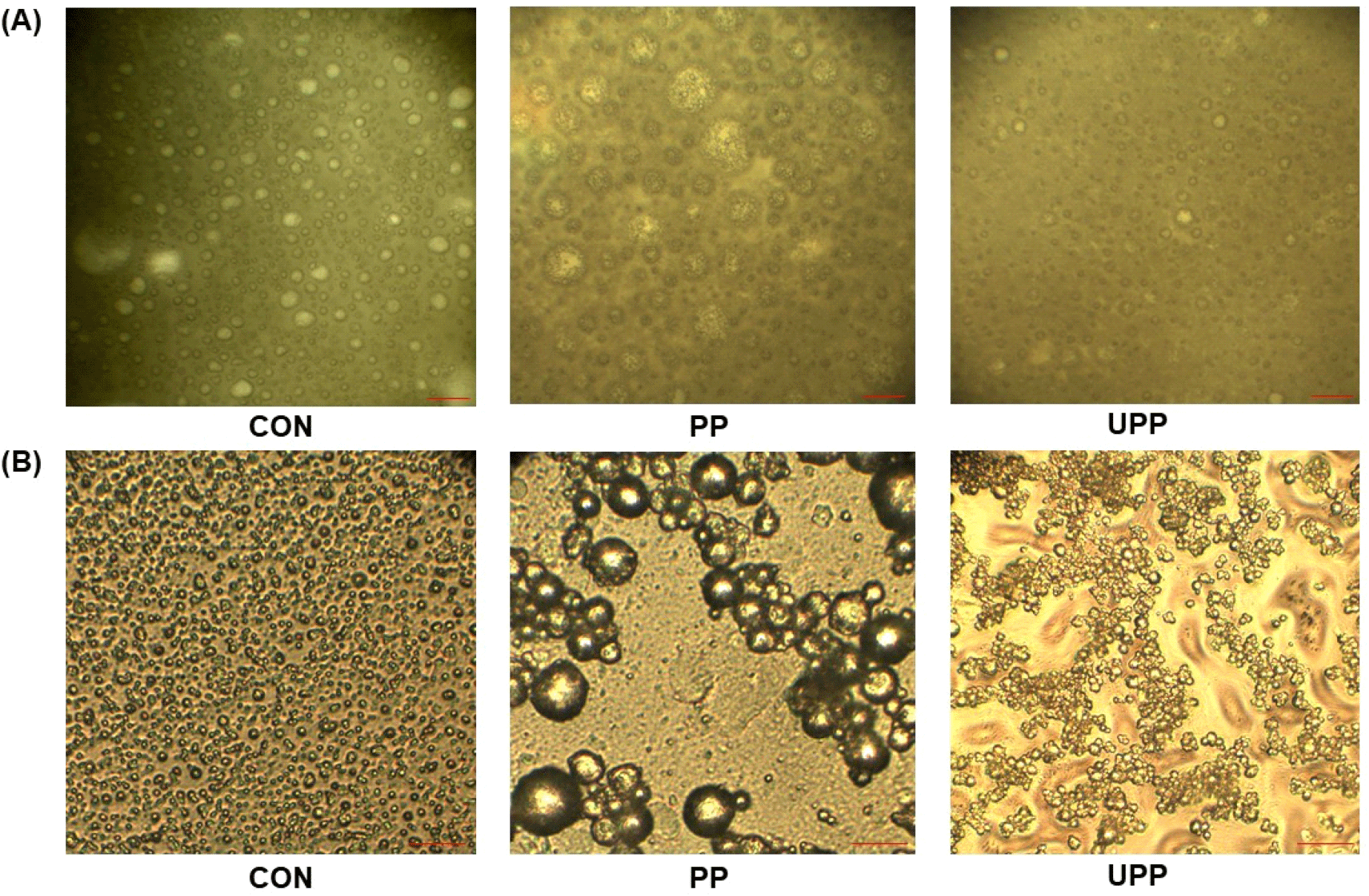
Table 4 shows the values measured to analyze the texture characteristics of whipped creams, including hardness, springiness, cohesiveness, and adhesiveness. Texture profile analysis is a widely used quality control tool in the food industry, and it is an essential for adapting whipped creams to foods affected by texture (Nguyen et al., 2015; Ningtyas et al., 2021). The hardness significantly decreased in the order of UPP, CON, and PP. If the whipped cream is not firm, it will not hold the weight of the topping when used in desserts and will run off and cannot be used for decoration, which is an important factor in the utilization of whipped cream (Goralchuk et al., 2020). Liu et al. (2021) reported that the higher degree of partial adhesion of fat globules, the higher the hardness as it forms a stronger network in the whipped cream, and it is effective in immobilizing air bubbles, so the results of this study showed a similar trend in fat destabilization and hardness. The springiness of whipped cream is a measure of its ability to return to its original condition after a deformation force is removed (Mohammadi et al., 2022). This property is influenced by the presence of proteins and fats. Ultrasonication was effective in reducing the size of protein particles and fat globules, resulting in improved springiness. Cohesiveness refers to the internal binding forces that make up whipped creams (Fu et al., 2020). Cohesiveness decreased significantly in the order of CON, UPP, and PP. Valdez-Hurtado et al. (2019) reported that the ultrasonication of proteins increased the cross-linking of the protein network, thereby resulting in a more cohesive gel. Therefore, ultrasonication increased the network of pea proteins in the whipped cream, resulting in a more cohesive whipped cream. Adhesiveness, a significant property of whipped cream, indicates its flowability or liquid state (Nguyen et al., 2015). The adhesion value decreased significantly in the order of UPP, CON, and PP. This difference may be attributed to variations in protein composition, which affects the overall protein surface properties (Ningtyas et al., 2021). Pea proteins’ surface hydrophobicity and zeta potential were enhanced by ultrasonication, two protein surface properties that positively influence emulsion stability (McCarthy et al., 2016; Qamar et al., 2019). It is worth noting that ultrasonication significantly improved the hardness and adhesiveness of whipped creams, which are essential textural properties.
The apparent viscosity of the whipped creams is shown in Fig. 3(A). A high apparent viscosity can prevent the escape of air bubbles and keep them stable. However, an excessively high viscosity cannot trap air during whipping, and a low viscosity cannot retain air bubbles (Wu et al., 2023; Yang et al., 2022). Apparent viscosity is an important factor in consumer acceptability and organoleptic properties, and is closely related to consumer preferences, including overall mouthfeel. A high viscosity indicates that the cream moves less in the mouth, and the resistance to movement can cause a sticky feeling on the palate (Fu et al., 2020; Laguna et al., 2021). The apparent viscosity tended to decrease for all whipped creams as the shear rate increased. This is because as the shear rate increases. As the shear rate increases, hydrodynamic forces also increase, causing particles to deform and eventually collapse. This results in a decrease in viscosity (Ningtyas et al., 2021). The viscosity of whipped creams at a low shear rate decreased in the order of CON, UPP, and PP. Yang et al. (2022) reported that ultrasonication can induce dissociation of protein subunits and promote the formation of fat globule networks with enhanced emulsification properties. This results in ultrasonicated pea protein emulsions with higher apparent viscosity. According to Allen et al. (2006), Table 4 shows that higher springiness, one of the results of the texture profile analysis, corresponds to greater resistance to shear force before breakdown. Therefore, springiness and apparent viscosity tend to be similar.
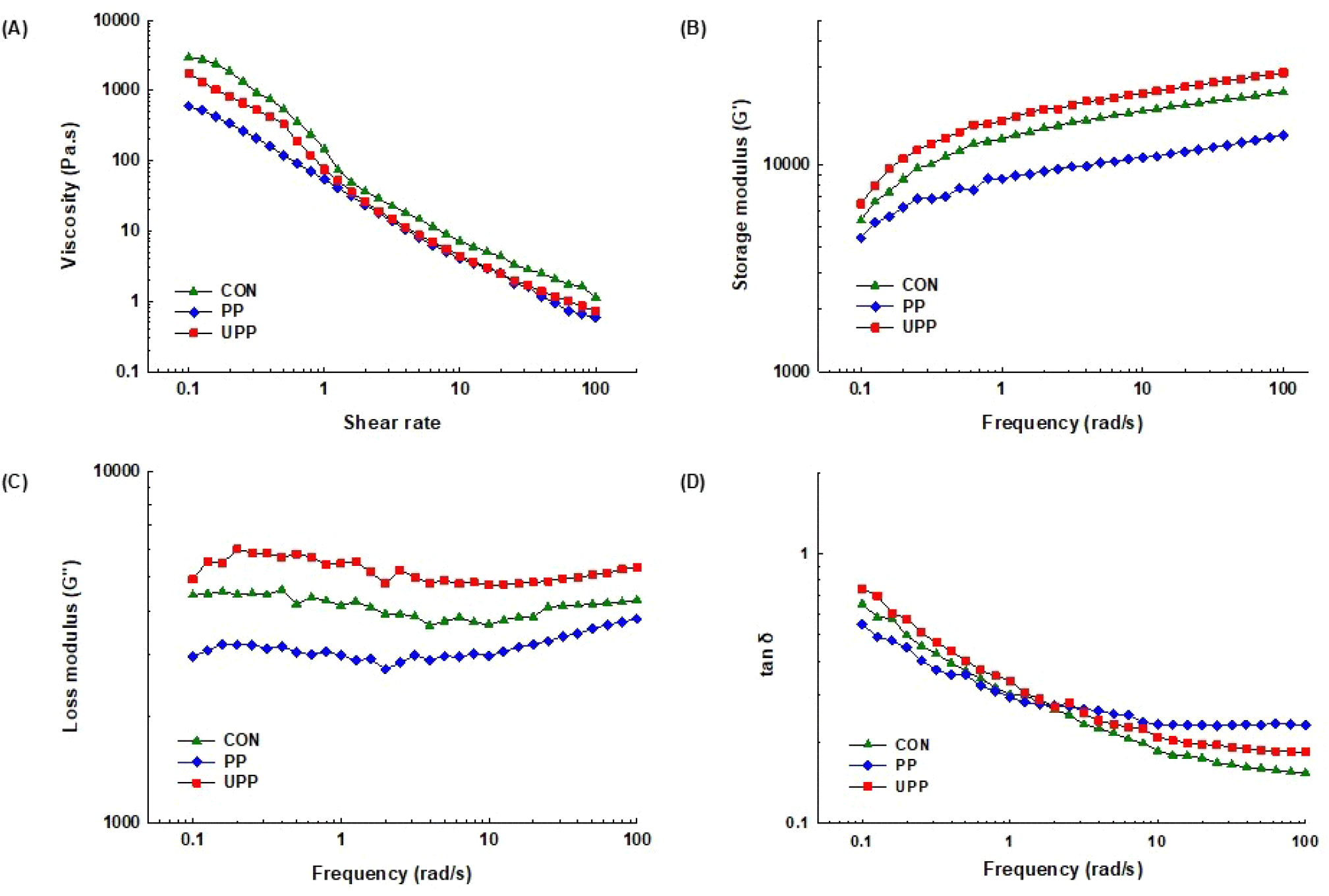
The storage modulus (G′) and loss modulus (G″) of the whipped creams is shown in Fig. 3 (B) and (C). The storage modulus (G′) and loss modulus (G″) represent the elastic and viscous behavior of the samples, respectively (Yang et al., 2022). The ratio of the storage modulus (G′) to the loss modulus (G″) is represented by tan δ (G″/G′). A value of tan δ<1 indicates prominent elastic behavior, while a value of tan δ>1 indicates prominent viscous behavior (Farahmandfar et al., 2017). The storage modulus increases as the frequency increases. UPP has the highest results, followed by CON and PP, and then decreases at all frequencies. Emulsions with higher fat contents expectedly exhibit higher storage modulus, which may explain the higher storage modulus of CON, which has a higher fat content than PP (Farahmandfar et al., 2017). However, UPP has the highest storage modulus due to its high fat destabilization, which forms a tight network structure (Wu et al., 2023). The loss modulus, similar to the storage modulus and apparent viscosity, is higher than that of PP because ultrasonication induced protein loosening in the protein solution phase, resulting in a stiffer network structure (Yang et al., 2022). The tan δ values shown in Fig. 3(D) indicate that all samples have tan δ<1, suggesting that both dairy and plant-based pea protein whipped creams exhibit solid-like behavior (Yang et al., 2022). These properties are consistent with the rheological properties of previously investigated whipped creams (Farahmandfar et al., 2017; Long et al., 2016; Seo and Yoo, 2022). Despite the lack of fat content compared with CON, ultrasonication resulted in the formation of a more robust network than CON, indicating that ultrasonication not only had a positive effect on the viscoelastic behavior of whipped creams but also resulted in more robust foam.
The results of the sensory evaluation are presented in Fig. 4. The results indicate that CON scored highest in appearance, color, and flavor, followed by UPP and PP. The firmness of whipped creams, evaluated as an element to assess the hardness of whipped creams, decreased significantly in the order of UPP, PP and CON. The TPA results in Table 4 show a similar trend to that of hardness. Additionally, UPP with higher fat destabilization indicated higher firmness because higher fat destabilization can increase partial fat adhesion to form a firm fat network. The overall acceptability was significantly lower in the order of CON, UPP, and PP.
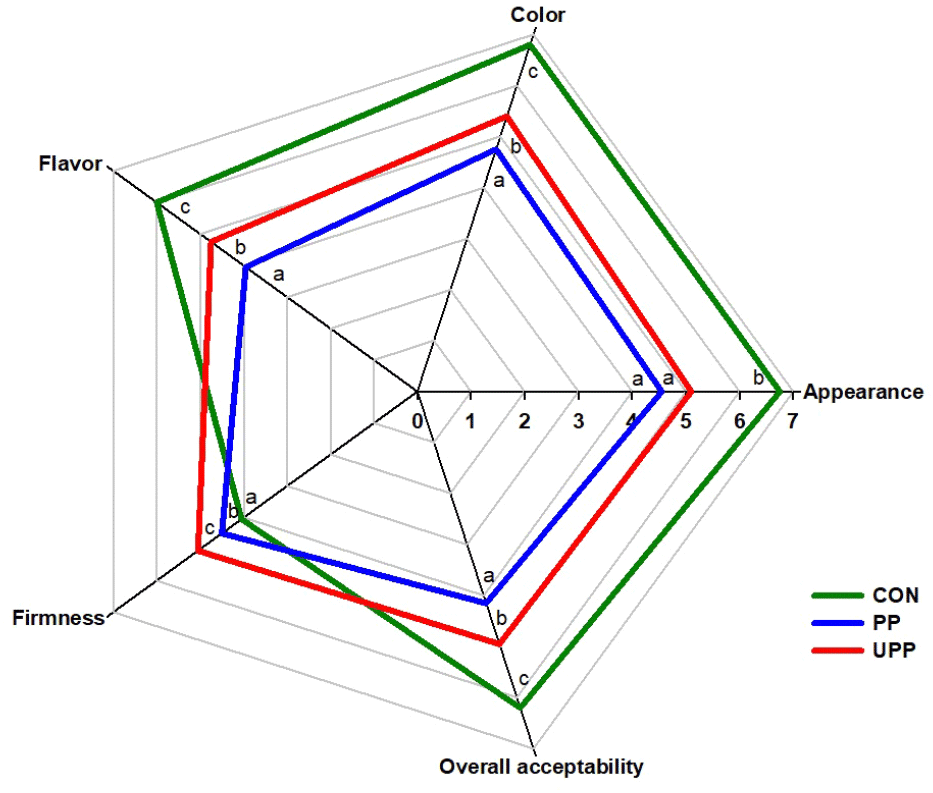
This is consistent with previous research that has shown that color, flavor, and appearance are influential factors in sensory analysis (Chambers and Koppel, 2013; Santagiuliana et al., 2019; Sipos et al., 2021). The results suggest that ultrasonication is a useful processing method for sensory superior whipped creams, as UPP was significantly higher than PP in terms of color, flavor, and overall acceptability among the plant-based pea protein whipped creams.
4. Conclusions
This study analyzes the quality characteristics of plant-based whipped creams using ultrasonicated pea proteins and their potential as a replacement for dairy whipped creams. The chromaticity of whipped creams was affected by the fat globule size, with UPP having a higher b* value than PP, and UPP showed the best results due to the effect of ultrasonication on overrun, foam drainage and fat destabilization, which are significant factors in whipped creams. Moreover, the fat globule size was reduced in UPP compared with PP. However, CON had the smallest size, confirming the effect of ultrasonication as observed using optical microscopy. With respect to the rheological properties of whipped creams, ultrasonication improved hardness and adhesion and had a positive effect on apparent viscosity and viscoelastic properties. The sensory evaluation indicated that UPP had lower overall acceptability scores than CON but higher scores than PP. This confirms that ultrasonication is a significant treatment method for achieving sensory superiority in whipped creams. The results suggest that plant-based whipped creams made with ultrasonicated pea proteins have potential as an alternative to dairy whipped creams. This study can be used as a source of quality characteristics of plant-based whipped creams and as a method to improve the utilization of pea proteins through ultrasonication as an emulsifier and foaming agent.