1. Introduction
In Korea, sweet potato soju was first produced in 1834, as documented in Jongjeobo (Seo, 1834), and in 1835, in Imwon Gyeongjeji (Seo, 1835). However, it did not gain widespread popularity, and it wasn’t until the 2010s that it began to be produced domestically. Interest in using sweet potatoes for soju production started to grow, and as of 2024, six types of sweet potato soju products have emerged (Shin, 2024). The import volume of distilled soju, including sweet potato soju, increased from 1,714 kL in 2019 to 4,905 kL in 2023, representing a 2.8-fold increase (Korea Agro-Fisheries & Food Trade Corporation, 2022). The characteristic aromatic components of sweet potato soju are monoterpene alcohols that exist as glycosides in sweet potatoes, resulting in a very weak aroma in raw sweet potatoes (Wang and Kays, 2000). During mash production, the heat applied during steaming and the action of β-glucosidase from koji during fermentation release geraniol and nerol, generating a characteristic aroma (Ohta et al., 1990). Some of the monoterpene alcohols released into the mash are converted to citronellol during fermentation; during distillation, they transform into linalool and α-terpineol due to heat and acids (Ohta et al., 1991), contributing to the distinctive aroma of sweet potato soju.
Typically, sweet potato soju is produced using a two-step fermentation process. In the first step, rice, water, and yeast are fermented, followed by the addition of steamed sweet potatoes in the second step for further fermentation before atmospheric distillation (Yoshizaki et al., 2011). Most distillation equipment in Korea employs indirect heating by supplying steam to the distillation jacket, which applies heat to the mash. However, the high viscosity of sweet potatoes causes considerable resistance to convection during distillation. Consequently, the temperature in areas where steam enters can increase, causing the mash to burn and produce off-flavors, leading to poor quality. In Japan, a method that injects steam directly into the mash is employed (Abe and Gomi, 2007); however, this type of equipment has not yet been implemented in Korea, creating production challenges.
Previous studies have demonstrated that it is possible to produce distilled soju without contamination by using enzymes instead of traditional rice preparation methods and by grinding raw rice rather than steaming it (Kwon et al., 2023; Kwon et al., 2024). This method reduces the equipment and labor required to prepare rice mash. Additionally, preliminary experiments indicated that using raw sweet potatoes instead of steamed ones did not significantly affect alcohol productivity while reducing viscosity, allowing the use of existing distillation equipment. Based on these findings, we hypothesized that sweet potato soju could be produced using only β-glucosidase to release the saccharification enzymes and monoterpene alcohol components. Applying reduced-pressure distillation could also minimize off-flavor development due to heat (Yi et al., 2010), which is likely to positively impact quality. In this study, distilled sweet potato soju was fermented under reduced pressure using enzymes, and the characteristics of the resulting soju were examined.
2. Materials and methods
The sweet potatoes used in this study were sourced from the Yesan region in Chungcheongnam-do, and the rice was purchased from Gangwon Province, specifically the Shindongjin variety. The starch for the alcohol mash was acquired from Korea Fermentation Co. Ltd. (Hwaseong, Korea). The enzymes included glucoamylase (Diazyme® X4) and α-amylase (Spezyme Fred), while β-glucosidase was purchased from Bision Biochem Co. (Seoul, Korea). Fermivin (DSM Food Specialties, Heerlen, Netherlands) was used as the yeast.
The compositions of materials used for brewing are presented in Table 1. The experimental groups were divided into two main categories: those using microorganisms (white koji) and those using enzymes (amylase + β-glucosidase) groups. In the microbial group, water and dry yeast were added to white koji to initiate the first fermentation. In the enzymatic group, polished rice was coarsely ground to less than 1.5 mm using a roller mill (Kyungchang, Seoul, Korea), then mixed with hot water at approximately 70°C and stirred at 950 rpm in a water bath at 68°C (HS-100T, DAIHAN Scientific Co., Ltd., Wonju, Korea). When the internal temperature reached 68°C, α-amylase and glucoamylase were added, and the mixture was stirred for 12 h for saccharification. After cooling the saccharified liquid to 30°C, yeast was added to initiate fermentation. Each treatment was fermented at 30°C for 5 days with daily stirring. Upon completion of the first fermentation, each treatment was divided into equal portions, and steamed or non-steamed sweet potatoes and water were added to initiate the second fermentation. The sweet potatoes were steamed at 100°C for 1 h, cooled to room temperature, and crushed. Non-steamed sweet potatoes were crushed using a blender (SHMF-3450S, HANIL ELECTRIC, Seoul, Korea). In the enzymatic hydrolysis treatment, α-amylase and glucoamylase were added based on preliminary experimental results, followed by the addition of β-glucosidase to release monoterpene alcohols. The second fermentation was conducted at 30°C for 7 days with daily stirring. During fermentation, weight was measured daily to calculate the cumulative weight loss.
The mash was distilled under reduced pressure at 110 mmHg using a rotary evaporator (R-114, BÜCHI Labortechnik AG, Flawil, Switzerland). The apparatus was equipped with a receiving flask and a measurable dropping funnel. The water bath temperature was set to 80°C, and the rotation speed was adjusted to 50 rpm for distillation. The mash was placed in the evaporation flask, and after applying a vacuum, the flask was immersed in the water bath to initiate distillation. Distillation was stopped when the distillate reached 40% (v/v) of the mash, and the distillate was collected. The distillation yield was calculated as the percentage of the recovered pure alcohol content relative to the pure alcohol content of the mash.
The physicochemical composition of the alcohol mash was analyzed according to the National Tax Service Liquor Analysis Regulations (National Tax Service Liquors License Support Center, 2014). To measure the alcohol content, 70 mL of distilled water was mixed with 100 mL of each sample and distilled. Approximately 80 mL of the distillate was collected and diluted to 100 mL with distilled water. The alcohol content was measured using an alcohol meter (DMA 101, Anton Paar Co., Graz, Austria).
The pH was measured in triplicate using 10 mL of the sample and a pH meter (Orion Star A214, Thermo Scientific Co., Waltham, MA, USA) with three repetitions.
Total acidity was measured by taking 10 mL of the sample, adding a mixed indicator 0.2 g Bromothymol Blue (Sigma Chemical Co., St. Louis, MO, USA) and 0.1 g Neutral Red (Sigma Chemical Co.) dissolved in 300 mL of 95% ethyl alcohol (Samchun, Seoul, Korea), and neutralizing with 0.1 N NaOH (Samchun), then converting it into citric acid.
For amino acid analysis, 10 mL of the sample was mixed with the indicator (Bromothymol Blue and Neutral Red) and neutralized with 0.1 N NaOH until the sample turned olive green. Then, 5 mL of neutral formalin solution (Biosesang, Yongin, Korea) was added. The sample was titrated with 0.1 N NaOH until it turned pink, and the values were converted to glycine content.
The free amino nitrogen (FAN) content was measured using the ninhydrin method with glycine (Sigma Aldrich Co.). The soluble solid content (°Brix) was measured using a refractometer (PAL-1, ATAGO®, Tokyo, Japan) (Horwitz and Latimer, 2011).
Reducing sugars were quantified using the Somogyi method and expressed as glucose content (Hatanaka and Kobara, 1980).
For free sugars and organic acid analysis, samples were centrifuged at 4°C (CR22N; Eppendorf Himac Technologies Co., Ltd., Tokyo, Japan). The supernatant was collected, filtered through a 0.45-μm syringe filter (Nylon, Sartorius AG), and used for analysis. Free sugar analysis was conducted using HPLC (e-2695, Waters Co., Milford, MA, USA) with a Shodex Asahipak NH2P-50 4E column (4.6×250 mm, Showa Denko, Tokyo, Japan). The mobile phase consisted of 75% acetonitrile (Mallinckrodt Baker Inc., Phillipsburg, NJ, USA) with a flow rate of 1.0 mL/min, a column oven temperature of 30°C, and an injection volume of 10 μL detected by an RI detector (2414, Waters Co.).
Organic acids were analyzed using the post-column method. HPLC (e-2695, Waters Co.) was connected to Pump B (S2100; Sykam GmbH, Eresing, Germany) for organic acid analysis, using a TSKgel ODS-100V column (4.6×250 mm, 5 μm, Tosoh, Tokyo, Japan) linked with a Hypersil GOLD C18 column (4.6×250 mm, Thermo Scientific Co.). The mobile phase of Pump A used 8 mM perchloric acid with a flow rate of 0.8 mL/min and a column oven temperature of 40°C. The eluent from the column reacted with the mobile phase of Pump B (0.2 mM bromothymol blue (Sigma Chemical Co.), 15 mM Na2HPO4 (Sigma Chemical Co.), and 7 mM NaOH and was detected at UV 440 nm. The flow rate of Pump B was set to 0.8 mL/min.
For enzyme activity measurement, the coenzyme solution was prepared by centrifuging the mash and saccharified liquid samples (CR22N, Eppendorf Himac Technologies Co., Ltd.) at 1,000 ×g for 10 min at 4°C. The supernatant was then collected and filtered (PVDF, 0.45 μm) before use.
The saccharification power was measured using a Kikkoman Saccharification Power Quantification Kit (60212, Kikkoman Co., Tokyo, Japan). The substrate, 4-nitrophenyl O-α-D-glucopyranosyl-(1→4)-β-D-glucopyranoside (G2-PNP), reacted with glucoamylase and α-glucosidase to produce G1-β4-nitrophenol (PNP), which was then broken down by the enzyme solution containing β-glucosidase, resulting in the release of the chromogenic compound PNP for activity measurement (Imai et al., 1996). A reaction mixture containing 0.5 mL each of substrate and enzyme solution was prepared and distributed into test tubes, then preheated at 37°C for 5 min. After adding 0.1 mL of the coenzyme solution, the mixture was stirred and reacted at 37°C for 10 min. The reaction was stopped by adding 2.0 mL of a stopping solution, and the absorbance of the released PNP was measured at 400 nm. For the blank, 1 mL of the reaction mixture was heated for 15 min before adding 2.0 mL of the stopping solution and 0.1 mL of the coenzyme solution, and the absorbance was measured at 400 nm. This absorbance value was used to calculate the Es and Eb values. For α-glucosidase, the substrate, 4-nitrophenyl-β-glucoside (PNPG), was acted upon solely by α-glucosidase to measure the released PNP. A 2.0 mL substrate solution was preheated at 37°C for 5 min, then 0.1 mL of the coenzyme solution was added and stirred before reacting at 37°C for 10 min. After adding 1.0 mL of the reaction solution, absorbance was measured at 400 nm. The enzymatic activity was calculated using a formula provided by the manufacturer:
where E2s is the α-glucosidase absorbance of a sample, E2b is the α-glucosidase absorbance of a sample, and Df is the dilution factor.
The α-amylase activity was measured using the Kikkoman α-amylase quantification kit (model no. 60213, Kikkoman Co.). The synthetic substrate, 2-chloro-4-nitrophenyl 65-azido-65-deoxy-β-maltopentaoside (N3-G5-β-CNP), was used, and the activity was assessed based on the release of the chromogenic compound 2-chloro-4-nitrophenol (CNP) by the action of α-amylase (Shirokane et al., 1996a). The reaction mixture was prepared by mixing 0.5 mL of each substrate solution and enzyme solution, which was then distributed into test tubes and preheated at 37°C for 5 min. After adding 0.1 mL of the coenzyme solution and stirring, the mixture was incubated at 37°C for 10 min. The reaction was stopped by adding 2 mL of stopping solution, and the absorbance of the released CNP was measured at 400 nm using a UV spectrophotometer (U-2900; HITACHI Co., Tokyo, Japan). For the blank, 1 mL of the reaction mixture containing 0.5 mL each of substrate and enzyme solution was heated for 15 min before adding 2 mL of stopping solution and 0.1 mL of coenzyme solution. The absorbance was measured in the same manner. Enzyme activity was calculated using the following formula, provided by the manufacturer, which indicates the volume (mL) of 1% starch solution that 1 g of enzyme could decompose at 40°C over 30 min (Shirokane et al., 1996b):
where Es is the absorbance of the sample, Eb is the absorbance of the blank, and Df is the dilution factor.
For the analysis of aroma components, distilled spirits with alcohol content adjusted to 25% using water were used. Six milliliters of dichloromethane (Sigma Aldrich Co.) were added to 30 mL of the soju sample. The lower layer was collected and filtered through a 0.45-μm syringe filter (Nylon, Sartorius AG) (Kishimoto et al., 2006).
Volatile aroma components were analyzed using a gas chromatograph (Nexis GC-2030; Shimadzu Co., Kyoto, Japan) connected to a gas chromatography/mass spectrometry (GC/MS) ISQ single quadrupole mass spectrometer (Thermo Fisher Scientific Inc.) using a Trace 1,310 gas chromatograph. The column used was a fused silica capillary column (30 m×0.32 mm×0.25 μm film thickness, NukolTM; Supelco, Bellefonte Co., PA, USA). The column oven temperature was programmed to start at 50°C for 5 min, followed by an increase of 3°C per min to 200°C for 5 min. The carrier gas (N2) flow rate was set to 24.2 cm/sec (linear velocity) with a split ratio of 20:1. The injector temperature was set to 250°C, and the detector was set to 280°C. Standard reagents for the analysis were obtained from Sigma-Aldrich Co., and quantification was performed using an external standard method.
For GC/MS analysis, a fused silica capillary column (60 m×0.32 mm×0.25 μm film thickness, SUPELCOWAXTM 10; Supelco Co.) was utilized. The column oven temperature was set to 45°C for 5 min, then increased by 3°C/min to 220°C for 5 min. Volatile aroma components were ionized using the electron impact ionization (EI) method. The GC/MS analysis conditions included an ionization voltage of 70 eV and a mass spectral scan range of 50-500 m/z. Helium was used as the carrier gas at a flow rate of 1.0 mL/min, and 1 μL of volatile aroma components was injected with a split ratio of 1:10. Standard reagents for the analysis were obtained from Sigma-Aldrich Co., and quantification was performed using an external standard method.
Statistical analyses were performed using IBM SPSS Statistics for Windows, version 12.0 (Chicago, IL, USA). Analysis of variance (ANOVA) was used to identify significant differences among samples, which were further evaluated using Duncan’s multiple range test at p<0.05. PCA was also performed to visually represent variations in the aroma components of sweet potato soju based on the treatment methods.
3. Results and discussion
The composition of the initial fermentation mash for white koji and rice saccharification is detailed in Table 2. The white koji treatment exhibited a pH of 3.19, total acidity of 1.67% citric acid, total amino acid content of 0.26% glycine, a urea amino nitrogen content of 3,130.90 mg/L, soluble solids at 11.87 °Brix, and reducing sugars at 7.54%. In contrast, the rice saccharification had an initial pH of 6.13, total acidity of 0.08% citric acid, total amino acid content of 0.06% glycine, FAN at 781.44 mg/L, soluble solids at 24.37 °Brix, and reducing sugars at 19.75%. The total free sugar content was approximately 21.4%, with glucose and maltose being the predominant sugars at 16.8% and 4.3%, respectively, followed by isomaltose and fructose.
Fig. 1 illustrates the changes in cumulative weight loss during the fermentation period for rice saccharification (CO2 release). The cumulative weight loss for rice saccharification was 5.0 g on day 1, 63.2 g on day 3, and a total of 115.2 g over 5 days. This was higher than the weight loss observed in the white koji mash, which was 1.3 g on day 1, 63.4 g on day 3, and 84.8 g over the same period. This difference is likely due to the higher fermentable free sugar content in rice saccharification (21.4%) compared to the white koji treatment (7.6%), indicating a 2.7-fold increase (Table 2). As shown in Table 3, after the first fermentation, the free sugar content was 0.3% for rice saccharification and 5.3% for the white koji treatment, reflecting lower residual sugar in rice saccharification and higher carbon source consumption. The alcohol content after the first fermentation was 16.32% for rice saccharification, similar to 16.17% for white koji mash, suggesting that carbon source consumption did not directly correlate with alcohol production. The pH, total acidity, and FAN levels for rice saccharification (Table 3) were 4.14, 0.30% citric acid, and 172.02 mg/L, respectively, differing from those of white koji mash (3.54, 1.78% citric acid, and 868.68 mg/L). The differences in pH and total acidity are attributed to the citric acid in white koji (Futagami, 2022) (Table 2), while the difference in FAN content likely results from the absence of protein-degrading enzymes in rice saccharification. The white koji mash contained acidic protease and carboxypeptidase activities (Sugimoto et al., 2012), leading to increased FAN levels. Consequently, the total amino acids in the saccharified rice and white koji mashes were 0.14% and 0.39% glycine, respectively, indicating that rice saccharification had a 2.8-fold lower amino acid content, with a 2.0-fold decrease in soluble solid content.
Fig. 2 shows the cumulative weight loss during the fermentation period of the first mash (CO2 release), comparing steamed versus non-steamed sweet potatoes. Weight loss from steamed sweet potatoes was over 30% higher than from non-steamed sweet potatoes throughout the fermentation period. Sweet potatoes contain β-amylase, which converts starch to maltose as the temperature rises during steaming (Kaplan et al., 2016). This suggests that the initial fermentation rate would be faster for steamed sweet potatoes compared to non-steamed ones. However, since the starch content of sweet potatoes was the same, we anticipated that if resistant starch in non-steamed sweet potatoes were broken down, final alcohol production would be similar. The general components, free sugars, and enzymatic activities of the second mash after fermentation are presented in Table 4. The residual enzyme activity in the fermented white koji and enzyme mash ranged from 0.86 to 1.26 U/mL for white koji and 0.55 to 0.72 U/mL for the enzyme treatment, indicating higher saccharification potential in the white koji. However, the alcohol content was higher in the enzyme treatment, ranging from 13.20% to 16.10%, compared to 10.74% to 13.76% for the white koji. The total free sugars were 2.2% to 6.3% for white koji and 2.4% to 4.6% for the enzyme treatment, suggesting a higher absorption of fermentable sugars in the enzyme treatment.
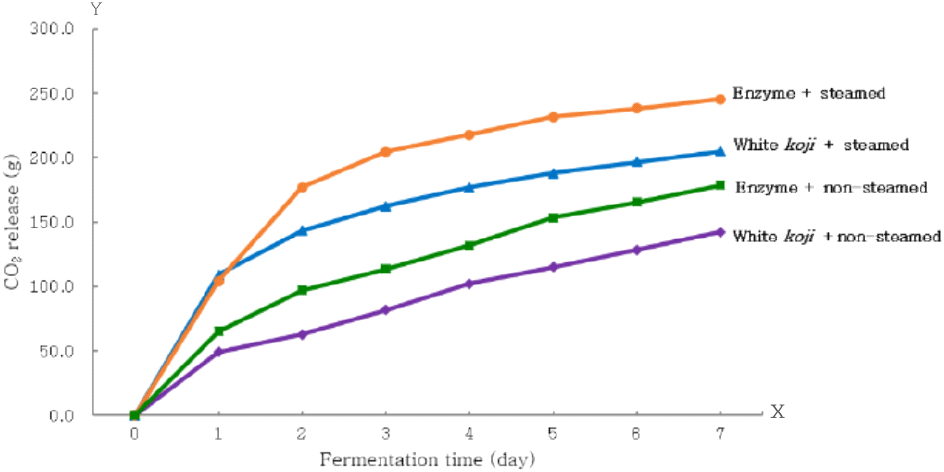
Regarding alcohol content based on whether sweet potatoes were steamed, the alcohol content was 13.76% for white koji with steamed sweet potatoes and 10.74% for non-steamed sweet potatoes, indicating a 1.3-fold lower alcohol content in non-steamed sweet potatoes. In the enzyme treatment, the alcohol content was 16.10% for steamed sweet potatoes and 13.20% for non-steamed sweet potatoes, showing a 1.2-fold lower alcohol productivity in non-steamed sweet potatoes. The reducing sugar content in the white koji mash with steamed sweet potatoes was 3.18%, compared to 2.73% with non-steamed sweet potatoes, indicating similar absorption of fermentable sugars by yeast. The residual enzyme activity in the non-steamed treatment showed α-amylase activity was 4.2 times higher and saccharification ability was 1.5 times higher. Overall, the lower starch breakdown capacity of non-steamed sweet potatoes appeared to negatively affect alcohol production. Therefore, extending the fermentation period beyond the 7 days used in this experiment may be necessary for the non-steamed sweet potato treatment to allow sufficient starch breakdown.
In summary, under the experimental conditions, the enzyme treatment method increased alcohol productivity by approximately 17% for steamed sweet potatoes and 23% for non-steamed sweet potatoes compared to the traditional white koji method. This increase can be attributed to the higher total acidity (0.57%) in white koji mash, which, along with rising alcohol concentrations, delayed the fermentation rate (Fig. 2), resulting in higher free sugar content and lower alcohol productivity.
The alcohol content of the distillate obtained from the second mash after vacuum distillation is listed in Table 5. The alcohol content of the distillate was 31.89% for white koji with steamed sweet potatoes, 25.09% for white koji with non-steamed sweet potatoes, 39.26% for enzyme-treated steamed sweet potatoes, and 28.42% for enzyme-treated non-steamed sweet potatoes. The distillation efficiencies, which indicate the ratio of recovered pure alcohol in the distillate to the total pure alcohol in the mash for each treatment, were 91.98%, 92.09%, 96.33%, and 83.98%, respectively. Enzyme-treated steamed sweet potatoes, which had the highest alcohol content in the second mash (Table 4), exhibited the highest distillation efficiency. However, the correlation coefficient between the alcohol content of the second mash and distillation efficiency was 0.389 (p=0.611), indicating a weak relationship.
The aromatic compounds in sweet potato soju, after adjusting the alcohol concentration to 25% by mixing with water, are presented in Table 6. A total of 54 aromatic compounds were detected in the 65 analyzed samples, including 14 alcohols, 16 esters, eight fatty acids, six terpenes, three aldehydes, two alkanes, two sulfides, three ketones, and one pyrazine.
Peak no. | Compounds | Identification | Quantificationions (m/z) | RI | Microbial (White Koji) |
Enzymatic (Amylase+β-glucosidase) |
Threshold References (mg/L) |
|||
---|---|---|---|---|---|---|---|---|---|---|
Steamed | Non-steamed | Steamed | Non-steamed | |||||||
Alcohols (14) | ||||||||||
5 | 1-Propanol(P) | MS | 59, 60 | 1029 | 46.67±5.511)B | 81.82±23.71A2) | 15.18±3.17C | 25.91±5.08BC | 950 | Oishi et al. (2013) |
9 | Isobutyl alcohol(B) | MS | 74, 56 | 1078 | 100.54±9.45BC | 196.50±41.38A | 61.82±12.30C | 119.33±20.44B | 150 | Oishi et al. (2013) |
14 | 1-Butanol | MS | 15, 24 | 1132 | 0.66±0.07B | 0.68±0.18B | 0.99±0.19AB | 1.17±0.21A | 280 | Oishi et al. (2013) |
17 | Isoamyl alcohol(A) | MS | 55, 70 | 1197 | 190.06±14.70B | 249.12±41.51A | 191.90±26.73B | 221.44±26.40A | 33 | Oishi et al. (2013) |
22 | 1-Hexanol | MS | 56, 55 | 1332 | 0.07±0.01B | 0.07±0.02B | 0.08±0.02B | 0.46±0.08A | 8 | Guth (1997) |
5 | 1-Propanol(P) | MS | 59, 60 | 1029 | 46.67±5.51B | 81.82±23.71A | 15.18±3.17C | 25.91±5.08BC | 950 | Oishi et al. (2013) |
25 | cis-3-Hexen-1-ol | MS | 67, 82 | 1375 | 0.03±0.00A | 0.05±0.01A | 0.04±0.01A | 0.04±0.01A | ||
27 | 2,3-Butanediol | FID | - | 1427 | 3.81±0.01A | 3.15±0.02C | 3.15±0.03C | 3.33±0.01B | ||
36 | Furfuryl alcohol | MS | 98, 97 | 1636 | 0.02±0.00B | 0.03±0.01A | 0.01±0.00B | 0.01±0.00C | ||
49 | Benzyl alcohol | MS | 108, 79 | 1862 | 0.05±0.01B | 0.10±0.02A | 0.04±0.01B | 0.09±0.02A | ||
50 | 2-Phenylethyl alcohol | MS | 91, 92 | 1869 | 35.34±1.73B | 50.72±6.62A | 42.34±5.58AB | 49.73±5.31A | 10 | Guth (1997) |
59 | 1-Tetradecanol | MS | 83, 69 | 2157 | 0.04±0.00A | 0.04±0.01A | 0.04±0.01A | 0.04±0.01A | ||
60 | p-Vinylguaiacol | MS | 50, 135 | 2184 | 2.93±0.33A | 3.46±1.09A | 0.41±0.08B | 0.30±0.07B | 0.04 | Chen et al. (2013) |
62 | Hexadecan-2-ol | MS | 72, 57 | 2223 | 0.16±0.06A | 0.17±0.09A | 0.14±0.05A | 0.13±0.05A | ||
Sum | 427.05±37.39B | 667.73±138.38A | 331.32±51.35B | 447.89±62.77B | ||||||
P+B+A | 337.27±29.66 | 527.44±106.60 | 268.90±42.20 | 366.68±51.92 | ||||||
A/P | 4.07±3.17 | 3.04±1.96 | 12.64±0.57 | 8.54±1.02 | ||||||
A/B | 1.89±0.75 | 1.26±0.39 | 3.10±0.23 | 1.85±0.34 | ||||||
B/P | 2.15±1.17 | 2.40±1.20 | 4.07±0.33 | 4.60±0.86 | ||||||
Esters (18) | ||||||||||
3 | Ethyl acetate | FID | 70, 61 | 894 | 158.22±0.73A | 126.98±9.37B | 73.04±5.81D | 100.27±2.36C | 7.5 | Guth (1997) |
4 | Ethyl isobutyrate | MS | 71, 88 | 953 | 0.22±0.02A | 0.29±0.07A | 0.31±0.04A | 0.28±0.06A | 0.0039 | Osafune et al. (2020) |
6 | Ethyl butyrate | MS | 71, 88 | 1033 | 1.00±0.11A | 1.02±0.34A | 0.92±0.21A | 0.91±0.20A | 0.026 | Osafune et al. (2020) |
11 | 2-Methylbutyl acetate | MS | 70, 55 | 1115 | 26.57±3.33A | 12.68±4.00B | 10.02±2.23C | 6.82±1.57D | ||
12 | Isoamyl acetate | MS | 70, 55 | 1116 | 20.19±2.18A | 11.10±2.62B | 9.35±1.46BC | 7.26±1.03C | 0.24 | Oishi et al. (2013) |
13 | Ethyl valerate | MS | 88, 85 | 1128 | ND3) | ND | ND | ND | ||
18 | Ethyl hexanoate | MS | 88, 99 | 1225 | 0.66±0.08A | 0.45±0.18AB | 0.35±0.08B | 0.29±0.07B | 0.015 | Oishi et al. (2013) |
20 | Ethyl heptanoate | MS | 88, 113 | 1315 | 0.01±0.00A | 0.01±0.00A | 0.01±0.00A | 0.01±0.00A | 0.002 | Guth (1997) |
23 | Ethyl lactate | FID | - | 1343 | 0.13±0.01B | ND | ND | 6.22±0.16A | 14 | Yin et al. (2020) |
26 | Ethyl octanoate | MS | 88, 101 | 1424 | 1.04±0.16A | 0.49±0.15B | 0.60±0.07B | 0.29±0.06B | 0.29 | Oishi et al. (2013) |
32 | Ethyl nonanoate | MS | 88, 101 | 1516 | 0.01±0.00B | 0.01±0.00B | 0.01±0.00B | 0.02±0.00A | ||
35 | Ethyl decanoate | MS | 57, 71 | 1627 | 0.60±0.05A | 0.18±0.05C | 0.42±0.09B | 0.09±0.02C | 0.56 | Oishi et al. (2013) |
43 | Methyl salicylate | MS | 120, 152 | 1765 | 0.56±0.06A | 0.87±0.30A | 0.83±0.19A | 0.86±0.20A | ||
44 | 2-Phenethyl acetate | MS | 91, 164 | 1771 | 0.02±0.00B | 0.12±0.03A | 0.02±0.01B | 0.11±0.02A | 0.25 | Guth (1997) |
48 | Ethyl dodecanoate | MS | 88, 101 | 1831 | 0.07±0.00B | 0.04±0.01C | 0.11±0.03A | 0.01±0.00C | 3.5 | Rahayu et al. (2017) |
55 | 2-Phenylethyl isovalerate | MS | 104, 105 | 1978 | ND | ND | ND | ND | ||
56 | Ethyl myristate | MS | 88, 101 | 2038 | 0.12±0.01BC | 0.31±0.10A | 0.21±0.05AB | 0.08±0.02C | 0.5 | Yin et al. (2020) |
63 | Ethyl palmitate | MS | 88, 101 | 2243 | 2.89±0.25B | 8.34±2.75A | 2.85±0.63B | 2.01±0.57B | 14 | Yin et al. (2020) |
Sum | 212.31±6.99A | 162.89±19.97B | 99.05±10.90D | 125.53±6.32C | ||||||
Fatty Acids (8) | ||||||||||
37 | Butyric acid | FID | 1653 | 1.07±0.01B | 1.06±0.03B | 1.30±0.04A | 0.98±0.02C | 10 | Guth (1997) | |
39 | Isovaleric acid | FID | 1678 | 0.87±0.12A | 0.79±0.02A | 0.76±0.02A | 0.82±0.02A | |||
42 | Valeric acid | FID | 1755 | 1.04±0.01AB | 0.78±0.21B | 0.95±0.26AB | 1.14±0.03A | |||
51 | Hexanoic acid | FID | 1855 | 0.59±0.01A | 0.46±0.04B | 0.46±0.03B | 0.50±0.01B | 3 | Chen et al. (2013) | |
54 | Heptanoic acid | FID | 1973 | 1.62±0.06A | 1.44±0.09AB | 1.51±0.11AB | 1.42±0.10B | |||
57 | Octanoic acid | FID | 2079 | 0.48±0.25A | 0.42±0.28A | 0.35±0.08A | 0.36±0.07A | 0.5a | Chen et al. (2013) | |
61 | Nonanoic acid | FID | 2176 | 0.66±0.01A | 0.57±0.02B | 0.28±0.03C | 0.28±0.01C | |||
64 | Decanoic acid | FID | 2293 | 0.51±0.01A | 0.47±0.02B | 0.30±0.01D | 0.34±0.01C | 15 | Guth (1997) | |
Sum | 6.84±0.48A | 5.99±0.71A | 5.91±0.58A | 5.84±0.27A | ||||||
Terpenes (6) | ||||||||||
15 | Myrcene | MS | 93, 69 | 1154 | 0.02±0.00B | 0.02±0.00BC | 0.03±0.01A | 0.01±0.00C | ||
33 | Linalool | MS | 71, 93 | 1532 | 0.03±0.00C | 0.09±0.02BC | 0.14±0.03B | 0.30±0.06A | 0.0066 | Osafune et al. (2020) |
38 | α-Terpineol | MS | 59, 93 | 1683 | 0.02±0.00B | 0.03±0.01B | 0.04±0.01B | 0.20±0.04A | 2.9 | Osafune et al. (2020) |
41 | β-Citronellol | MS | 69, 81 | 1749 | 0.05±0.02B | 0.06±0.02B | 0.07±0.01AB | 0.09±0.02A | 0.27 | Osafune et al. (2020) |
45 | Nerol | MS | 69, 93 | 1783 | 0.04±0.01B | 0.12±0.03A | 0.14±0.01A | 0.15±0.03A | 1.1 | Osafune et al. (2020) |
47 | Geraniol | MS | 69, 68 | 1829 | 0.01±0.00B | 0.06±0.01A | 0.03±0.00B | 0.07±0.02A | 0.026 | Osafune et al. (2020) |
Sum | 0.17±0.03C | 0.38±0.09B | 0.45±0.07B | 0.82±0.17A | ||||||
Aldehydes (5) | ||||||||||
2 | Butyraldehyde | MS | 72, 57 | 879 | 0.31±0.03A | 0.42±0.11A | 0.39±0.08A | 0.40±0.07A | ||
10 | Hexanal | MS | 56, 57 | 1079 | 10.82±1.25BC | 20.51±4.32A | 7.15±0.76C | 12.69±2.32B | 0.02 | Osafune et al. (2020) |
29 | Furfural | MS | 95, 96 | 1448 | 1.94±0.01C | 1.94±0.01B | 1.95±0.01A | 1.95±0.01B | 15 | Oishi et al. (2013) |
31 | Benzaldehyde | MS | 105, 106 | 1513 | ND | ND | ND | ND | 4.203 | Wang et al. (2023) |
58 | cis-3-Hexenal | FID | - | 2086 | ND | ND | ND | ND | ||
Sum | 13.07±1.29BC | 22.87±4.44A | 9.49±0.85C | 15.04±2.40B | ||||||
Alkanes (2) | ||||||||||
16 | Dodecane | MS | 57, 71 | 1200 | 38.47±3.60B | 50.61±10.27A | 37.87±3.72B | 40.99±2.87AB | ||
34 | Hexadecane | MS | 57, 71 | 1600 | 0.06±0.01A | 0.09±0.03A | 0.10±0.02A | 0.08±0.02A | ||
Sum | 38.53±3.61B | 50.70±10.30A | 37.97±3.74B | 41.07±2.89AB | ||||||
Sulfides (5) | ||||||||||
1 | Dimethyl sulfide | MS | 62, 61 | <780 | 2.00±0.01A | 2.01±0.03A | 2.01±0.01A | 2.01±0.01A | 0.01 | Guth (1997) |
8 | Dimethyl disulfide | MS | 93, 78 | 1064 | ND | ND | ND | ND | 0.0091 | Wang et al. (2023) |
24 | Dimethyl trisulfide | MS | 126, 79 | 1367 | ND | ND | ND | ND | 0.0002 | Guth (1997) |
28 | Methional | MS | 104, 76 | 1444 | ND | ND | ND | ND | ||
40 | Methionol | MS | 106, 61 | 1701 | 0.51±0.05AB | 0.73±0.20A | 0.29±0.06B | 0.68±0.14A | ||
Sum | 2.51±0.06AB | 2.74±0.23A | 2.30±0.07B | 2.69±0.15A | ||||||
Ketones (5) | ||||||||||
7 | 2,3-Pentanedione | MS | 57, 100 | 1042 | 0.22±0.02B | 0.28±0.07A | 0.23±0.04A | 0.22±0.04B | ||
21 | 6-Methyl-5-hepten-2-one | MS | 108, 55 | 1327 | ND | ND | ND | ND | ||
46 | Damascenone | MS | 69, 121 | 1808 | 0.01±0.01AB | 0.02±0.00A | 0.01±0.00B | 0.01±0.00AB | 0.0083 | Osafune et al. (2020) |
52 | β-Ionone | MS | 177, 178 | 1929 | ND | ND | ND | ND | ||
53 | Maltol | MS | 126, 71 | 1952 | 0.28±0.01A | 0.45±0.14A | 0.40±0.10A | 0.38±0.09A | ||
Sum | 0.51±0.04A | 0.75±0.21A | 0.64±0.14A | 0.60±0.13A | ||||||
Pyrazines (2) | ||||||||||
19 | 2-Methylpyrazine | MS | 94, 67 | 1245 | ND | 0.01±0.00B | 0.01±0.00A | 0.01±0.00B | 10.5 | Chen et al. (2013) |
30 | 2,3,5,6-Tetramethylpyrazine | MS | 136, 57 | 1461 | ND | ND | ND | ND | 80.073 | Wang et al. (2023) |
Sum | ND | 0.01±0.00 | 0.01±0.00 | 0.01±0.00 |
The total alcohol content was 427.05 mg/L for white koji with steamed sweet potatoes, 667.73 mg/L for white koji with non-steamed sweet potatoes, 331.32 mg/L for enzyme-treated steamed sweet potatoes, and 447.89 mg/L for enzyme-treated non-steamed sweet potatoes. The total alcohol content was higher in the non-steamed sweet potatoes than in the steamed sweet potatoes, primarily due to the higher levels of 1-propanol (P), isobutyl alcohol (B), and isoamyl alcohol (A) in the non-steamed sweet potato soju. The A/P ratio was 3.04-4.07 for white koji with sweet potatoes compared to 8.54-12.64 for enzyme-treated sweet potato soju, indicating a greater than twofold difference. The B/P ratio also demonstrated a similar trend, with values of 2.15-2.40 for white koji sweet potato soju and 4.07-4.60 for enzyme-treated sweet potato soju. This difference can be attributed to the lower concentration of 1-propanol (P) in enzyme-treated sweet potato soju (15.18-25.91 mg/L) compared to white koji sweet potato soju (46.67-81.82 mg/L).
1-Propanol (P) is produced by the metabolism of glycine and threonine (Wang et al., 2021), isobutyl alcohol (B) from valine, and isoamyl alcohol (A) from leucine (Guymon, 1964). The difference in the FAN content of the mash (Table 4) is thought to contribute to the variation in high-quality alcohol production. In contrast, the content of 2-phenylethyl alcohol, known for its rose-like aroma, was higher in enzyme-treated sweet potato soju (42.34-49.73 mg/L) compared to white koji sweet potato soju (35.34-50.72 mg/L).
In terms of processing methods, the total alcohol content in soju made from non-steamed sweet potatoes was 1.5 times higher in the white koji treatment and 1.3 times higher in the enzyme treatment than in soju made from steamed sweet potatoes.
The total amount of esters was higher in white koji sweet potato soju (162.89-212.31 mg/L) compared to enzyme-treated sweet potato soju (99.05-125.53 mg/L). The content of ethyl acetate in white koji sweet potato soju was 126.98-158.22 mg/L, whereas, in enzyme-treated sweet potato soju, it was 73.04-100.27 mg/L, indicating that the difference in ethyl acetate content was a significant factor contributing to the difference in total ester content. Ethyl acetate has a relatively low detection threshold of 7.5 mg/L and affects the sensory quality of soju (Yuan et al., 2024). In addition, isoamyl acetate (known for its banana aroma) (Tomimoto et al., 2020), ethyl hexanoate (known for its pineapple aroma) (Wang et al., 2019), and ethyl octanoate (known for its sweet and fruity aroma) (Komes et al., 2006) were lower in enzyme-based sweet potato soju than in traditional sweet potato soju made using white koji.
Esters are produced by the action of enzymes, such as esterase and alcohol acetyltransferase, on higher alcohols. Because the content of higher alcohols was lower in enzyme-based sweet potato soju, it can be inferred that the content of these ester components was also lower. The contents of 2-phenethyl acetate, an ester of 2-phenylethyl alcohol, were similar. Regarding the difference between steamed and non-steamed sweet potatoes, the treatment using steamed sweet potatoes in traditional rice soju was 1.3 times higher; however, in the enzyme-based treatment using non-steamed sweet potatoes, it was also 1.3 times higher, showing a contrasting result.
In fatty acids, the total content in traditional sweet potato soju ranged from 5.99 to 6.84 mg/L, while the enzyme-based sweet potato soju ranged from 5.84 to 5.91 mg/L, with no significant differences observed among the eight detected components.
The total terpene content was 0.17-0.38 mg/L in white koji sweet potato soju and 0.45-0.82 mg/L in enzyme-based sweet potato soju, indicating that the latter had more than double the content. Specifically, when comparing steamed and non-steamed sweet potatoes, the use of non-steamed sweet potatoes showed values 2.2 times higher in traditional soju and 1.8 times higher in enzyme-based soju. The terpene content of traditional non-steamed sweet potato soju and enzyme non-steamed sweet potato soju included linalool (0.09, 0.30 mg/L), α-terpineol (0.03, 0.20 mg/L), β-citronellol (0.06, 0.09 mg/L), nerol (0.12, 0.15 mg/L), and geraniol (0.06, 0.07 mg/L), with higher values in enzyme non-steamed sweet potato soju. As hypothesized in this experiment, the addition of β-glucosidase likely resulted in the release of mono-terpene alcohols, increasing the terpene content via yeast metabolism. In particular, the use of non-steamed sweet potatoes most likely helped to prevent the loss of volatile terpenes during the steaming process (Kim et al., 2008), resulting in a higher terpene content compared to steamed sweet potatoes. Additionally, no significant differences were observed in the alkane, sulfide, ketone, or pyrazine contents among the samples.
To confirm the variability of the aroma components in sweet potato soju according to the different treatment methods, principal component analysis (PCA) was conducted, as shown in Fig. 3. The explanatory power of PC1 (first principal component) was 38.32%, while that of PC2 (second principal component) was 51.80%, collectively accounting for a high explanatory power of 90.12%. In PC1, white koji sweet potato soju appeared in the first (non-steamed sweet potato) and second quadrants (steamed sweet potato), and enzyme-based sweet potato soju appeared in the third (steamed sweet potato) and fourth quadrants (non-steamed sweet potato). In terms of the relationships with aroma components, traditional sweet potato soju showed a high correlation with the aromatic components alcohols, aldehydes, alkanes, and sulfides, while enzyme-treated non-steamed sweet potato soju exhibited a high correlation with terpenes and ketones.
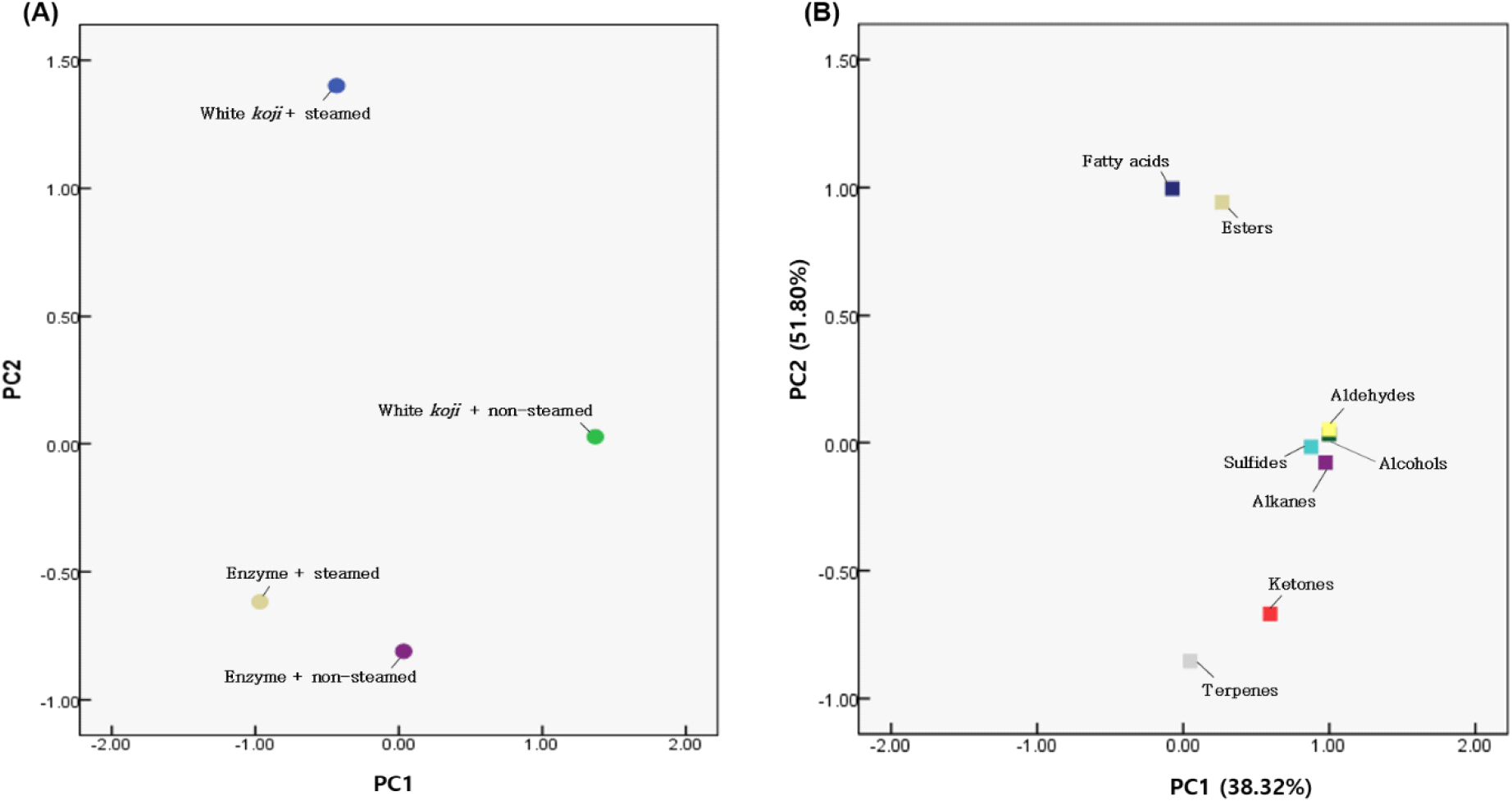
In summary, the method of producing sweet potato soju using enzymes can increase the alcohol content compared to the white koji method, and the addition of β-glucosidase can enhance the terpene content, which is a positive outcome. Although using non-steamed sweet potatoes may lower alcohol production, it can prevent the loss of terpenes during the processing of sweet potatoes, thereby increasing their soju content.
4. Conclusions
During sweet potato soju production, enzymes are utilized to enhance manufacturing convenience and reduce costs. Herein, the characteristics of this method are compared with those of traditional methods using white koji. The cumulative weight reduction of the fermentation mash using enzymes is over 30% higher than that of the white koji method, and alcohol productivity improves by 17-23%. A total of 54 aromatic compounds are detected in sweet potato soju, including 14 alcohols, 16 esters, eight fatty acids, six terpenes, three aldehydes, two alkanes, two sulfides, three ketones, and one pyrazine. The total amount of alcohols (aroma compounds) is 427.05 mg/L for white koji-steamed sweet potato, 667.73 mg/L for non-steamed sweet potato, 331.32 mg/L for enzyme-steamed sweet potato, and 447.89 mg/L for enzyme non-steamed sweet potato, showing that the non-steamed sweet potato has a higher total alcohol content compared to the steamed version. The total amount of esters is higher in white koji sweet potato soju, ranging from 162.89 to 212.31 mg/L, compared to 99.05-125.53 mg/L for enzyme sweet potato soju. The total fatty acids are 5.99-6.84 mg/L for white koji sweet potato soju and 5.84-5.91 mg/L for enzyme sweet potato soju. The total terpenes are found to be higher in enzyme sweet potato soju, ranging from 0.45 to 0.82 mg/L, compared to 0.17-0.38 mg/L for white koji sweet potato soju, indicating a more than twofold increase. PCA demonstrates an explanatory power of over 90% of the total variance. White koji non-steamed sweet potato soju shows a high correlation with aromatic compounds, such as alcohols, aldehydes, alkanes, and sulfides. In contrast, enzyme-treated non-steamed sweet potato soju exhibits a strong correlation with terpenes and ketones. These results indicate that the method of producing sweet potato soju using enzymes increases the alcohol content of the mash compared to the white koji method. Furthermore, the addition of β-glucosidase appears to positively enhance the terpene content in sweet potato soju.